ARKU Maschinenbau GmbH has introduced the EdgeBreaker 6000 deburring machine, designed to meet the requirements of laser job shops. Its vacuum conveyor belt and rotary brushes enable extremely uniform edge rounding, the company reports. Other processing modules include a grinding belt for deburring and a finishing unit for surface finishing.
ARKU Wizard software can be added to the machine to adjust processing settings automatically during deburring. The operator enters a few parameters—burr size, material type and thickness, desired edge rounding after deburring—and further information comes from the automatic wear measurement of the rotary brushes. The software then calculates the appropriate tools and the correct machine settings. Application Of Deburring Machine

Processing speed is optimized and the material removal during edge rounding is reduced to the necessary level. This helps decrease processing time and tool wear.
The FABRICATOR is North America's leading magazine for the metal forming and fabricating industry. The magazine delivers the news, technical articles, and case histories that enable fabricators to do their jobs more efficiently. The FABRICATOR has served the industry since 1970.
Easily access valuable industry resources now with full access to the digital edition of The FABRICATOR.
Easily access valuable industry resources now with full access to the digital edition of The WELDER.
Easily access valuable industry resources now with full access to the digital edition of The Tube & Pipe Journal.
Easily access valuable industry resources now with full access to the digital edition of The Fabricator en Español.
This week, Jessica Considine – a welder, fabricator, and artist based in the Detroit-area – joins The Fabricator...
© 2023 FMA Communications, Inc. All rights reserved.
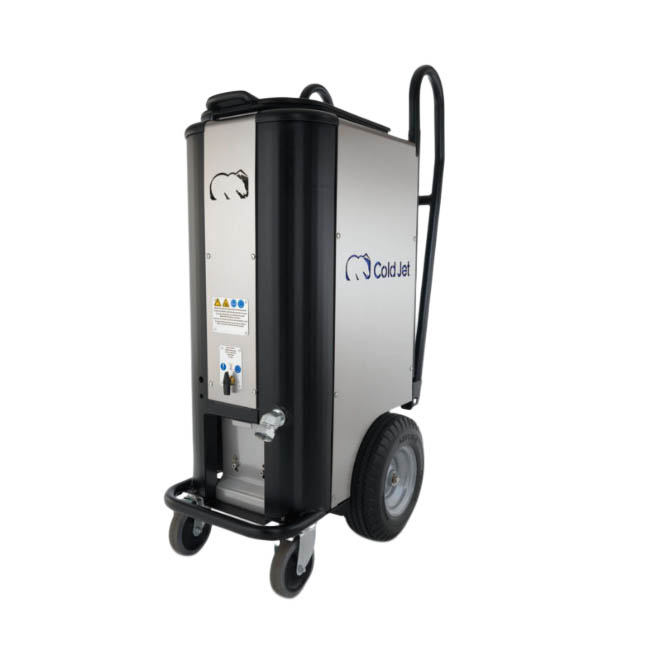
Deburring Solutions Not yet registered? Sign up