Thank you for visiting nature.com. You are using a browser version with limited support for CSS. To obtain the best experience, we recommend you use a more up to date browser (or turn off compatibility mode in Internet Explorer). In the meantime, to ensure continued support, we are displaying the site without styles and JavaScript.
Scientific Reports volume 13, Article number: 6632 (2023 ) Cite this article Indoor Cycle Exercise Bikes

Whereas cardiopulmonary responses are well understood in endurance training, they are rarely described in strength training. This cross-over study examined acute cardiopulmonary responses in strength training. Fourteen healthy male strength training-experienced participants (age 24.5 ± 2.9 years; BMI 24.1 ± 2.0 kg/m2) were randomly assigned into three strength training sessions (three sets of ten repetitions) with different intensities (50%, 62,5%, and 75% of the 3-Repetition Maximum) of squats in a smith machine. Cardiopulmonary (impedance cardiography, ergo-spirometry) responses were continuously monitored. During exercise period, heart rate (HR 143 ± 16 vs. 132 ± 15 vs. 129 ± 18 bpm, respectively; p < 0.01; η2p 0.54) and cardiac output (CO: 16.7 ± 3.7 vs. 14.3 ± 2.5 vs. 13.6 ± 2.4 l/min, respectively; p < 0.01; η2p 0.56) were higher at 75% of 3-RM compared to those at the other intensities. We noted similar stroke volume (SV: p = 0.08; η2p 0.18) and end-diastolic volume (EDV: p = 0.49). Ventilation (VE) was higher at 75% compared to 62.5% and 50% (44.0 ± 8.0 vs. 39.6 ± 10.4 vs. 37.6 ± 7.7 l/min, respectively; p < 0.01; η2p 0.56). Respiration rate (RR; p = .16; η2p 0.13), tidal volume (VT: p = 0.41; η2p 0.07) and oxygen uptake (VO2: p = 0.11; η2p 0.16) did not differ between intensities. High systolic and diastolic blood pressure were evident (62.5% 3-RM 197 ± 22.4/108.8 ± 13.4 mmHG). During the post-exercise period (60 s), SV, CO, VE, VO2, and VCO2 were higher (p < 0.01) than during the exercise period, and the pulmonary parameters differed markedly between intensities (VE p < 0.01; RR p < 0.01; VT p = 0.02; VO2 p < 0.01; VCO2 p < 0.01). Despite the differences in strength training intensity, the cardiopulmonary response reveals significant differences predominantly during the post-exercise period. Intensity-induced breath holding induces high blood pressure peaks and cardiopulmonary recovery effects after exercise.
Dominic A. Notter, Samuel Verges, … Christina M. Spengler
Nuno Manuel Frade de Sousa, Danilo Rodrigues Bertucci, … Diogo Mello da Rosa
Yan Wang, Zhiyuan Li, … Xiaoxiao Li
The preventive and rehabilitative effects of physical exercise have generally been well-studied1,2,3,4,5,6. Strength training (ST) is an essential part of the guidelines for physical activity for health maintenance and for chronic diseases or disabilities7.
The cellular response to ST is widely acknowledged, as are the associated hypertrophic effects of skeletal muscle8,9,10. With regard to morphological adaptations of the cardiac muscle, effects of ST are described as concentric hypertrophy (wall thickening), whereas endurance training induces eccentric hypertrophy (the internal dimensions increase, as does the thickness of the wall)11,12.
Not conclusively clarified and unlike endurance training, the acute hemodynamic adaptations (stroke volume and cardiac output) of strength exercises have rarely been described13. The stroke volume und cardiac output response during body-weight strength training (5 body-weight exercises; e.g. squats, push-ups, inverted rows, isometric exercises) seems lower than during endurance training (continuous cycling; 70% heart rate max)14. Resistance (heavy weight-lifting or isometric exercise) training-induced blood pressure increase, on the other hand, is extrem elevated at high intensities15,16. There is evidence that executing strength training (e.g. high load/low repetition or low load/high repetition) has a significant influence on the amount of cardiovascular exertion during and after exercise17,18. Moreover, the amount of exercised muscle mass (comparing different strength exercises) has an impact on cardiovascular response in strength training19.
However, strength training protocols differ with respect to working-muscle groups, modality (dynamic vs. isometric, body weight vs. device-supported), duration, and intensity. Whereby, the intensity and cumulative volume of strength training protocols exert a decisive influence on muscular adaptations20,21,22.
Although strength training has grown increasingly popular in recent decades and regular strength training’s long-term effects have been widely documented23,24,25,26,27, the acute cardiopulmonary response moreover is often only investigated by relying on standard parameters like heart rate and oxygen uptake. Hemodynamic parameters have been primarily analyzed in conjunction with endurance training28,29 and seldom in strength training, whereby the few studies differ regarding the comparison groups in intensity, number of repetitions17,18, or used muscle mass19. Gjovaag et al. compared high load/low repetition resistance training (RT) to low load/high repetition RT and found significant different blood pressure and cardiac output responses during and after exercise. Also, during different strength exercises, the involved muscle mass showed different hemodynamic responses19. However, to date, there has been no investigation comparing the acute hemodynamic response in standardized strength training with the same number of repetitions at different intensities. In addition, breath holding is a natural reflex triggered during resistance exercises when greater effort is required15,30, which is likely to affect cardiac parameters. Therefore, dose–response relationships between strength training intensity and cardiopulmonary effort are important for the practical application of RT in terms of adaptation and risk potential in healthy and diseased humans.
The aim of this randomized crossover study was, therefore, to investigate hemodynamic cardiopulmonary responses (stroke volume, cardiac output, cardiac work, end-expiratory gas concentrations) during and after strength training with different intensities (50%, 62.5%, and 75% of the 3-Repetition Maximum; 3-RM). Based on the known long-term effects of exercise training, we expect the strongest cardiopulmonary and vascular peripheral responses at 75% of the maximum load.
Our study was conducted in accordance with the latest version of the Declaration of Helsinki and approved by the Ethics Committee of the Medical Faculty of the University of Leipzig (272/21-ek). Written informed consent was obtained from all participants. The study group consisted of 14 healthy and strength training experienced men (Table 1). Exclusion criteria were cardiac, pulmonary, or inflammatory diseases, athletic inactivity, and orthopedic anomalies at the time of the examinations. In addition, participants had to be able to perform the strength exercises technically and conditionally. We calculated sample size (G*Power software; Heinrich-Heine-Universität Düsseldorf, Germany) according to the expected effect of different load intensities on stroke volume. In our preliminary study, stroke volume rose by 10 ± 16 ml (effect size d = 1.2) at 75% of 3-RM compared to 50% 3-RM. Based on a power of 0.8 with a two-sided paired test and an alpha of 0.05, a sample containing at least 12 participants was required.
After the initial pre-examination, all participants completed three experimental sessions involving standardized squats on a smith machine (Technogym Germany GmbH, Germany) over a three-week period. To standardize the training sessions, the individual three-repetition-maximum at 50%, 62.5% and 75% were used. The pre-examination included medical history, lifestyle questionnaire (physical activity, smoking, and alcohol consumption), incremental exercise test (IET), and bioelectrical impedance analysis (Bioimpedance Analyzer BIACORPUS RX 4004 M, MEDI CAL HealthCare GmbH, Germany). The participants performed an incremental exercise test (IET) to exhaustion to determine maximum power output (Pmax), cardiac and pulmonary maximum values.
All participants performed three load conditions on separate days at the same time with a break lasting at least 5 days to ensure adequate recovery. On the first strength training session, the individual 3-repetition maximum (3-RM) after a warm-up period was determined via the approximation method, which corresponds to the maximum amount of weight lifted in clean execution for three repetitions in the smith machine. The 3-RM tests followed the ACSM guidelines for 1-RM tests31. After 3-RM determination (mean: 95.1 ± 24.4 kg), participants were allowed to rest for 20 min to reduce potential fatigue effects. Thereafter, they engaged in the training session with 50% of 3-RM load. The training session was then repeated at 62.5% 3-RM and 75% 3-RM loads on separate days in random order. At an additional session, blood pressure was measured during single-armed squatting at 62.5% 3-RM applying the Riva-Rocci/Korotkoff method.
All participants took part in a warm up-period lasting 5 min on a bicycle ergometer (100 W; 75 rpm) at each training session, followed by one set of 10 repetitions without external load, five repetitions with 50% of the subsequent testing load and 3 repetitions with 75% of the subsequent load. For each training session three sets of 10 repetitions were completed for each load with a 4-min rest period between each set32,33 to ensure cardiopulmonary and metabolic recovery and to analyze the whole post-exercise period (Fig. 1). Squats were standardized via an individual knee flexion angle and with a timed execution target (2 s descending, 2 s ascending and 2 s hold at the top; i.e. six seconds per repetition). Each subject received visual and auditory feedback regarding correct task execution. Subjects were instructed to avoid any exercise training for 48 h before the sessions.
shows the timeline of measurements (A) execution and equipment (B). (A) The three load conditions (3 sets of 10 repetitions interspaced by a resting period of 4 min; intensities: 50%, 62.5% and 75% of 3-RM) were performed on separate days with a break lasting at least 5 days to ensure adequate recovery. The order of sessions 2 and 3 was randomized. On the first day, the individual 3-repetition maximum (3 RM) in the smith-machine back squat was determined. (B) Resting and post-exercise period = upright in a smith machine; exercise periods = ten repetitions within 60 s; Execution = 2 s descend, 2 s ascend and 2 s hold at the top; Ventilation and hemodynamic were recorded continuously; blood pressure measurement (only in post-exercise period): immediately after exercise, 1:30 min after exercise, and 2:30 after exercise; Blood pressure measurements during strength training sessions were not taken during the standardized strength training sessions because of interference in the repetition sequence and for safety reasons.
The IET was performed on a semi-recumbent ergometer (ergometrics 900, ergoline GmbH, Bitz, Germany) at a constant speed of 60–70 rpm. The test started with a 50 W load, which was increased by 15 W every minute until exhaustion occurred. The criteria for exhaustion were a cycling cadence below 60 revolutions per minute, a respiratory quotient above 1.1 and/or reaching the limit of perceived exertion. Each subject continued the test for an additional 5-min recovery period at a 25% load of Pmax. Spirometry (K4b, Cosmed, Italy), thoracic impedance (PhysioFlow, Manatec Biomedical, France), and an electrocardiogram (custo, BT300 custo GmbH, Germany) were synchronized and ran simultaneously during the entire time. Impedance cardiography employs disposable sensors on the neck and chest to measure electrical and impedance changes in the thorax. The change in impedance signal and its timing due to blood flow in the aorta are used to calculate hemodynamic parameters. Furthermore, blood pressure (BP) and rating of perceived exertion (RPE) were recorded every 3 min during the IET. The maximal load and cardiopulmonary parameters (IET 100%) at this level represent the reference for the maximum dynamic workload (Tables 2, 3).
The three strength training sessions in this study focused on mean exercise (three sets lasting 1 min), mean immediate post-exercise (1 min after three sets), and cumulated values (three sets with complete post-exercise period). Peak exercise and mean post-exercise values (4 min after three sets) are shown in the supplementary material.
Cardiac output (CO), stroke volume (SV), end-diastolic volume (EDV), ejections fraction (EF), and heart rate (HR) measured by impedance cardiography (sampling interval 10 s), maximum oxygen consumption (VO2max), end-tidal oxygen- and carbon dioxide-partial pressure (PetO2; PetCO2), respiratory rate (RR), tidal volume (VT) and minute ventilation (VE) measured by mobile spiroergometry and were monitored continuously at rest, during training, and after the training sessions. For impedance cardiography, six disposable sensors on the neck and chest are used to transmit and detect electrical and impedance changes in the thorax induced by the cardiac flow. The electrodes were applied in a standardized manner after skin preparation (peeling and disinfection) according to the manufacturer's instructions for each training session.
For editing purposes, the values were averaged at 10-s intervals. We calculated mean and peak values during the sets (60 s. excluding warm-up, rest and cool down), as well as mean and peak values during the resting phase (240 s.). For the comparison of the acute post-exercise period (Table 3), the mean value of the first 60 s. after each set was analyzed. We also collected heartbeats, CO, respiration cycles, VE, VO2, and VCO2 data to compare absolute values during the entire training sessions, including resting and exercise periods.
The arteriovenous oxygen difference (avDO2) was calculated using Fick's principle with avDO2 = oxygen uptake (V̇O2)/cardiac output (CO). Stroke work (SW) was measured in Joules (J) and calculated according to the formula SW = SV × MAP/7.534. Total peripheral resistance (TPR) was determined from heart rate corrected calculated mean arterial blood pressure (MAP = 1/3 × SBP + 2/3 × DBP) × (1 + (HR-60)/1000)35 and cardiac output (TPR = MAP/CO).
Blood pressure (BP) and rating of perceived exertion (RPE; from 1 to 10, if 10 was total exhaustion) were observed at rest, immediately after each set, and after 1.30 and 2.30 min of recovery. On a separate day, participants had to perform the squats in a single-arm position in an additional examination to enable blood pressure to be measured while exercising at 62.5% of the 3-RM. An experienced investigator took the blood pressure measurements with an upper arm cuff and a stethoscope. The subject did only one set of 10 repetitions with a slower repetition frequency, and blood pressure was measured indirectly during the eccentric movement between the seventh and tenth repetitions according to the method of Riva-Rocci. We took these measurements in 12 participants of the described study group. Decelerated repetitions of the squats allowed the investigator to move along with them and take the measurements with no interference.
All values are expressed as the means and standard deviation unless otherwise stated, and the significance level was defined as p < 0.05. Data were analyzed using Microsoft Office Excel® 2007 for Windows (Microsoft Corporation, Redmond, Washington, USA) and GraphPad Prism 9 for Windows (GraphPad Software Inc., California, USA). For distribution analysis, the D’Agostino-Pearson normality test was used. If normal distribution was evident, statistical comparisons between the intensity sessions were made using one-way repeated measures ANOVA with Tukey’s post hoc test for multiple comparisons. Otherwise, the nonparametric Friedman test and Dunn post-hoc test were used to compare the different training intensities. Within-group differences were made with a paired Student’s t-test.
All procedures described in this study will be performed in accordance with the ethical standards of the responsible committee on human experimentation (institutional and national) and with the principles of the Declaration of Helsinki of 1964 and its latest version. Written informed consent or its equivalent will be obtained from all patients. The protocol has been approved by the Ethics Committee of the Medical Faculty of the University of Leipzig (272/21-ek).
Maximum IET values are shown in Table 2. The participants achieved an average maximum power output of 291.1 ± 31.4 W corresponding to relative power of 3.7 ± 0.5 W/kg.
Baseline values were measured prior to each session (values not shown), and we observed no significant hemodynamic differences. Figures 2, 3 and 4 illustrate the time course of cardiac, pulmonary, and periphery responses across the three strength training sessions.
Graphs show the mean cardiac response (n = 14) during strength training sessions with (A) heart rate; (B) stroke volume, (C) cardiac output and (D) systolic—and diastolic blood pressure; Exercise blood pressure is only illustrated during 62.5% intensity.
Graphs show the mean pulmonary response (n = 14) during strength training sessions: (A) respiratory rate; (B) tidal volume, (C) minute ventilation and (D) oxygen uptake.
Graphs show the mean periphery and end-tidal course during strength training sessions with (A) Rating of perceived exertion; (B) Arteriovenous difference of oxygen (C) End-tidal oxygen-partial pressure, and (D) End-tidal carbon dioxide-partial pressure.
Table 2 shows comparisons of the mean values at the three strength training intensities.
The mean values of the three intensities revealed large differences in the cardiopulmonary response (Table 2). HR and CO showed significantly higher values during 75% intensity than at 50% or at 62.5% of the 3-RM. Ejection fraction (EF%) exhibited significantly higher values during exercise at 75% of the 3-RM than at 62.5% of the 3-RM (67.3 ± 6.3 vs. 64.2 ± 5.7%; p = 0.04). No differences in EF (%) were observed between 50% and 62.5% or 75% of the 3 RMs (64.9 ± 5.2 vs. 64.2 ± 5.7; p = 0.86; 64.9 ± 5.2 vs. 67.3 ± 6.3; p = 0.13).
VE was significantly higher at 75% 3-RM compared to the other two intensities. Our post-hoc comparison yielded significantly lower values in the PetCO2 parameter at 75% 3-RM than at 50% 3-RM (37.9 ± 2.32 vs. 38.8 ± 1.6; p = 0.04). PRE was significantly higher at 75% of the 3-RM than at 50% and 62.5% of the 3-RM.
Table 3 illustrates significant differences in cardiac (HR and CO) and pulmonary (VT, RR, VO2, and VCO2) parameters after the strength training (mean of one minute after three sets). Calculated avDO2 was the same at all intensities.
To compare the three training intensities, the HR, CO, VE, VO2, and VCO2 parameters were accumulated throughout the training duration (Table 4). Compared with 50% and 62.5%, 75% intensity revealed significantly higher O2 consumption, carbon dioxide production, cardiac output, breathing volume, and more breathing cycles as well as heartbeats.
Figure 2 shows mean responses for HR, CO, SV, and blood pressure time courses across the three strength training intensities. The intensities differed strongly in terms of the cardiopulmonary response with the exception of SV (Fig. 2 and Table 2). Figure 2 also shows that SV increases immediately after the exercise period. Exercise blood pressure is only illustrated during 62.5% of 3-RM intensity.
The course of ventilation parameters (RR; VT; VE and VO2) is shown in Fig. 3. We observed significant differences only in the VE value between intensities. RR tended to drop during squat execution and rise after finishing the exercise.
The RPE, calculated avDO2, and the end-tidal gas concentrations of O2 and CO2 are shown in Fig. 4. RPE during the intensities differed significantly, whereas the avDO2 course reveals no differences between intensities. Observing the end-tidal gas concentrations’ course, a substantial change in the exercise period becomes apparent. Normalization does not occur until the post-stress period (Fig. 4). We calculated differences between the exercise and post-exercise periods to illustrate the change in cardiopulmonary parameters (Table 5). SV and CO increased significantly during the post-exercise period compared to the exercise period. Pulmonary parameters (VE, VO2, VCO2, PetCO2) increased significantly after the end of exercise compared to the mean exercise-period values, as did the cardiac parameters.
Additional results, such as the peak values during the exercise periods and mean values of the entire post-exercise period are available in the supplement (Supplementary Tables 1 and 2).
The main finding of this randomized cross-over study was the specific post-exercise responses of cardiopulmonary parameters (SV, VE, VO2, PetO2, PetCO2). Due to the repetition-dependent breathing pattern with pressurized breathing and a rise in blood pressure during the exercise period, post-exercise cardiopulmonary responses revealed hyperventilation and an increase in stroke volume. As expected, an intensity-dependent cardiopulmonary (HR, CO, EF) response was evident during the exercise period. In contrast, other parameters revealed no intensity-dependent change (VO2, VT, RR, SV, EDV) during exercise.
During the exercise period, only VE, PetO2 and VCO2 differed between intensities. However, compared to the IET, VE values during the exercise period remained well below, as expected. Repetition-dependent breathing during the execution period seems to be a causal factor here. General recommendations suggest exhaling during the concentric phase of strength training and inhaling during the eccentric phase36. Similarly, in the present study, we specified the execution rhythm—a factor that strongly influenced the respiration pattern with additional pressurized breathing in the “time under tension” (Fig. 3). MacDougall et al. showed that the use of the Valsalva maneuver (VMs) during strength exercises is a natural reflex triggered during resistance exercises when greater effort is required, i.e. increasing proportionally to the work intensity30,37. Figure 3 shows the repetition-dependent breathing pattern during exercise. The RR decreases during strength exercise repetitions in all three conditions, and increases again after the load. According to the ventilation values, the PetO2 revealed highest values at 75% intensity of 3-RM. Hackett et al. compared strength training with five sets to strength training with two sets and showed that VE was higher and PetCO2 lower when the number of sets was higher38. Following Hackett et al., both PetO2 and PetCO2 parameters indicate comparatively higher alveolar ventilation at 75% of 3RM compared to the other intensities38. We hypothesized that 75% intensity compared to 62.5%, as well as 50% of the 3-RM, would lead to higher central command and peripheral afferent feedback (in group III/IV) in agreement with the significantly higher RPE and thus explain the higher VE during the higher workload39,40,41,42. In summary, the respiratory parameters show no differences due to the strength training-related repetition, with the exception of VE and VCO2, which reflect intensity-dependent effort.
During the post-exercise period, all pulmonary parameters exhibited significant intensity-dependent differences. Breathing regulation after the exercise period seems to be affected by the exercise-related breathing pattern. Therefore, the training-induced oxygen deficit and CO2 enrichment in blood were more pronounced during higher intensities. As a result, excessive post-exercise oxygen consumption (EPOC) is significantly higher at high intensities (75%) than at medium intensities (62.5%) and this in turn is higher than at low intensities (50%)43. This relationship was also evident in the VE characteristics (Table 3). The strong increase in pulmonary parameters during the post-exercise period further indicates execution-induced breath holding during high-intensity strength training. End-expiratory gas concentrations normalized after 1–2 min, thus explaining the afterload hyperventilation44.
In summary, an intermittent Valsalva maneuver during exercise has been observed at all three intensities30,36,37. Most of our participants’ pulmonary parameters, therefore, exhibited no intensity-dependent differences. Only the pulmonary response in VE was significantly stronger at high intensities (75%) than at low intensities (62.5% and 50%)14,38, which was particularly evident during the post-exercise period at all three intensities38,43. Nevertheless, during the entire training period, the cumulative parameters between intensities (Table 4) demonstrate a clear intensity-dependent difference, while correspondingly different degrees of the respiratory muscles’ long-term adaptation likely.
During the exercise period a significant intensity-related increase in HR and CO was evident (Tables 2 and 3; Fig. 2). SV tends to demonstrate an intensity-dependent increase (Tables 2 and 3). We hypothesize that pressurized breathing and increased TPR37,45 limit the rise in stroke volume during exercise and thus, depending on its intensity, both increased cardiac inotropy and chronotropy are responsible for elevated CO46. Filling pressures (EDV) did not significantly differ between intensities in our study, so that the observed increase in contractility (EF) at 75% intensity is not related to differences in EDV. Rather, increased contractility at 75% intensity appears to be the potential result of a rising heart rate (Bowditch "staircase") and or increased cardiac drive47,48.
The present results show that the HR and CO during exercise and during cumulative exercise and resting times was significantly higher at 75% intensity than at the two lower intensities (Table 4). Despite a significantly higher HR during exercise, EDV did not fall at 75% intensity. Thus, the significantly higher EF, as well as the tendency toward increased SV during 75% 3-RM strength training, argues for more afterload-compensating cardiac effort47. Therefore, we noticed a pronounced increase in CO induced by the force-frequency relationship (Bowditch effect) during 75% of 3RM, unlike at the other intensities48.
We recorded the blood pressure during exercise at 62.5% RM in follow-up examinations. The squats were done with one arm at a slowed repetition frequency. As doing the squats while measuring blood pressure was equivalent to the training sessions except for the lower speed, one can assume the assessed blood pressure values to be representative, with a tendency to higher values49. Because of the standardized repetitions (six seconds per repetition) and to ensure the safety of our participants, we were unable to measure the blood pressure during the main examinations. The blood pressure during exercise at 62.5% intensity revealed that both systolic and diastolic values increased substantially during exercise, unlike the resting or post-exercise values. Other investigations have also reported very high strength training-induced blood pressure levels15,16. It is well known that an increase in blood pressure depends on the intensity of exercise15. There is evidence that cardiac afterload plays a central role in myocardial function during high intensity exercise46,50. Figure 2 shows that SV increased only slightly during the exercise period due to the assumed high blood pressure. Once the exercise period is finished, the SV increases abruptly (Fig. 2 and Table 5). This seems to be attributable to the afterload blood pressure. According to Rowland et al.46, increasing contractility (EF%) and the heart rate during higher intensity (75% of 3-RM) compared with lower intensity (50% and 62.5% of 3-RM) could be the cardiac response to stronger peripheral resistance during strength exercise46. Greater cardiac exercise requirements are reflected in higher heart rates at 75% intensity of 3-RM and explain the higher CO and corresponding VO2.
In the post-exercise period SBP and DBP did not differ between intensities. This might relate to decreased vascular resistance (TPR) after strength exercise with higher intensity because of either higher flow-mediated and/or metabolic vasodilation51. The consistently significantly higher VCO2 values at 75% of 3RM intensity than at the lower loads (50% and 62.5% of 3RM) are a potential explanation for this.
Strength training does not seem to stimulate oxygen uptake substantially more than endurance training12,13. The acute cardiac response during strength training is known to be lower than during endurance training14, which probably explains the limited long-term effects of strength training on the cardiopulmonary system. However, the strength training in this previous study consisted of body-weight exercises, not equipment-based high intensity-strength training. High-intensity strength training involving equipment will likely elicit stronger cardiopulmonary responses. Aerobic exercise training is known to induce cardiovascular changes, including a functional increase in maximum cardiac output12. Cardiac adaptations are thus associated with improved contractility and an increase in blood volume, which raises the SV52,53,54. However, significant improvements in aerobic capacity have also been demonstrated in older adults through high- and low-intensity resistance training55,56.
To summarize: the cardiac parameters (unlike the pulmonary parameters due to pressurized breathing), reveal a more distinct intensity-dependent response during exercise. Significant increases in CO are evident during the post-exercise period, which is attributable to the lower blood pressure without load.
The sample size is small, and we selected only male and recreationally-active participants for this study to prevent compromising interference from possible gender differences in cardiopulmonary function, and muscle performance differences. Therefore, the interpretability and generalizability of the results is limited and only apply to a young male-only and healthy population. The main difficulty during our investigation was measuring blood pressure during strength exercises. For safety reasons (requiring a single-arm execution) and because of the repetition sequence’s influence, we were unable to take blood-pressure measurements during our participants’ strength sessions. We thus cannot quantify differences in blood pressure during exercise. Nevertheless, we did take single-arm blood pressure measurements (Riva-Rocci/Korotkoff) in 12 participants at an additional session during which the squats were performed at a slower repetition frequency (62.5% 3-RM). Cardiac parameters obtained via impedance cardiography may be overestimated using absolute values57. However, since intra-individual differences were compared, changes in these parameters were more relevant than the absolute values. Other working groups have carried out thoracic impedance cardiography to detect intra-individual changes in SV and CO28,58,59. Stroke volume and cardiac output measurements via impedance cardiography shows acceptable agreement compared with magnet resonance imaging and direct Fick or thermodilution methods57,60,61.
With this randomized cross-over study, we examined the acute hemodynamic response to standardized strength training at different intensities but of the same duration and muscle mass and during exercise and post-exercise periods. The cumulative cardiopulmonary response of exercise and post-exercise periods corresponds to the intensity differences. However, during the exercise period, a repetition-dependent breathing pattern was observed. Equipment-supported high-intensity strength training resulted in repetition-adjusted ventilation with breath holding and a markedly increase in blood pressure during the exercise period. Blood pressure dropped immediately after exercise, followed by a substantial increase in stroke volume during the immediate post-exercise period. The training-induced oxygen deficit and CO2 enrichment in blood is compensated by post-exercise hyperventilation. The post-exercise period in strength training thus differs from that in endurance training but is, however, probably associated with proven long-term cardiopulmonary adaptations in strength training. Nevertheless, the results of this study were conducted with healthy, recreationally-active and male individuals and are not generally transferable to other cohorts. In this context, high strength training intensity is of critical importance concerning specific cardiopulmonary adaptations, while pressurized breathing should be avoided and only moderate intensity should be used during rehabilitation.
The original contributions presented in the study are included in the article’s supplementary material; further inquiries can be directed to the corresponding author/s.
Cornelis, J., Beckers, P., Taeymans, J., Vrints, C. & Vissers, D. Comparing exercise training modalities in heart failure: A systematic review and meta-analysis. Int. J. Cardiol. 221, 867–876 (2016).
Haykowsky, M. J. et al. Meta-analysis of aerobic interval training on exercise capacity and systolic function in patients with heart failure and reduced ejection fractions. Am. J. Cardiol. 111, 1466–1469 (2013).
Parmenter, B. J., Dieberg, G. & Smart, N. A. Exercise training for management of peripheral arterial disease: A systematic review and meta-analysis. Sports Med. 45, 231–244 (2015).
Strasser, B., Siebert, U. & Schobersberger, W. Effects of resistance training on respiratory function in patients with chronic obstructive pulmonary disease: A systematic review and meta-analysis. Sleep Breath. 17, 217–226 (2013).
Streckmann, F. et al. Exercise intervention studies in patients with peripheral neuropathy: A systematic review. Sports Med. 44, 1289–1304 (2014).
Weston, K. S., Wisløff, U. & Coombes, J. S. High-intensity interval training in patients with lifestyle-induced cardiometabolic disease: A systematic review and meta-analysis. Br. J. Sports Med. 48, 1227–1234 (2014).
Garber, C. E. et al. American College of Sports Medicine position stand. Quantity and quality of exercise for developing and maintaining cardiorespiratory, musculoskeletal, and neuromotor fitness in apparently healthy adults: guidance for prescribing exercise. Med. Sci. Sports Exerc. 43, 1334–1359 (2011).
Evans, D. L. Cardiovascular adaptations to exercise and training. Vet. Clin. N. Am. Equine Pract. 1, 513–531 (1985).
Figueiredo, V. C. & McCarthy, J. J. Regulation of ribosome biogenesis in skeletal muscle hypertrophy. Physiol. Bethesda Md 34, 30–42 (2019).
Mesquita, P. H. C. et al. Skeletal muscle ribosome and mitochondrial biogenesis in response to different exercise training modalities. Front. Physiol. 12, 725866 (2021).
Article PubMed PubMed Central Google Scholar
Morganroth, J., Maron, B. J., Henry, W. L. & Epstein, S. E. Comparative left ventricular dimensions in trained athletes. Ann. Intern. Med. 82, 521–524 (1975).
Article CAS PubMed Google Scholar
Fagard, R. H. Impact of different sports and training on cardiac structure and function. Cardiol. Clin. 15, 397–412 (1997).
Article CAS PubMed Google Scholar
Spence, A. L. et al. A prospective randomised longitudinal MRI study of left ventricular adaptation to endurance and resistance exercise training in humans. J. Physiol. 589, 5443–5452 (2011).
Article CAS PubMed PubMed Central Google Scholar
Falz, R. et al. Acute cardiopulmonary responses to strength training, high-intensity interval training and moderate-intensity continuous training. Eur. J. Appl. Physiol. 119, 1513–1523 (2019).
MacDougall, J. D., Tuxen, D., Sale, D. G., Moroz, J. R. & Sutton, J. R. Arterial blood pressure response to heavy resistance exercise. J. Appl. Physiol. Bethesda Md 1985(58), 785–790 (1985).
Taylor, K. A., Wiles, J. D., Coleman, D. D., Sharma, R. & O’driscoll, J. M. Continuous cardiac autonomic and hemodynamic responses to isometric exercise. Med. Sci. Sports Exerc. 49, 1511–1519 (2017).
Perry, B. G. et al. Hemodynamic response to upright resistance exercise: Effect of load and repetition Med. Sci. Sports Exerc. 46(479), 487 (2014).
Gjovaag, T., Hjelmeland, A. K., Oygard, J. B., Vikne, H. & Mirtaheri, P. Acute hemodynamic and cardiovascular responses following resistance exercise to voluntary exhaustion. Effects of different loadings and exercise durations. J. Sports Med. Phys. Fitness 56, 616–623 (2016).
Matos-Santos, L., Farinatti, P., Borges, J., Massaferri, R. & Monteiro, W. Cardiovascular responses to resistance exercise performed with large and small muscle mass. Int. J. Sports Med. 38, 883–889 (2017).
Schoenfeld, B. J., Peterson, M. D., Ogborn, D., Contreras, B. & Sonmez, G. T. Effects of low- vs high-load resistance training on muscle strength and hypertrophy in well-trained men. J. Strength Cond. Res. 29, 2954–2963 (2015).
MacInnis, M. J. & Gibala, M. J. Physiological adaptations to interval training and the role of exercise intensity. J. Physiol. 595, 2915–2930 (2017).
Article CAS PubMed Google Scholar
Lixandrão, M. E. et al. Magnitude of muscle strength and mass adaptations between high-load resistance training versus low-load resistance training associated with blood-flow restriction: A systematic review and meta-analysis. Sports Med. Auckl. NZ 48, 361–378 (2018).
Bell, G. J., Syrotuik, D., Martin, T. P., Burnham, R. & Quinney, H. A. Effect of concurrent strength and endurance training on skeletal muscle properties and hormone concentrations in humans. Eur. J. Appl. Physiol. 81, 418–427 (2000).
Article CAS PubMed Google Scholar
Chilibeck, P. D., Syrotuik, D. G. & Bell, G. J. The effect of concurrent endurance and strength training on quantitative estimates of subsarcolemmal and intermyofibrillar mitochondria. Int. J. Sports Med. 23, 33–39 (2002).
Article CAS PubMed Google Scholar
Toigo, M. & Boutellier, U. New fundamental resistance exercise determinants of molecular and cellular muscle adaptations. Eur. J. Appl. Physiol. 97, 643–663 (2006).
Hansen, D. et al. Continuous low- to moderate-intensity exercise training is as effective as moderate- to high-intensity exercise training at lowering blood HbA(1c) in obese type 2 diabetes patients. Diabetologia 52, 1789–1797 (2009).
Article CAS PubMed PubMed Central Google Scholar
O’Bryan, S. J. et al. Progressive resistance training for concomitant increases in muscle strength and bone mineral density in older adults: A systematic review and meta-analysis. Sports Med. Auckl. NZ https://doi.org/10.1007/s40279-022-01675-2 (2022).
Lepretre, P., Koralsztein, J. & Billat, V. L. Effect of exercise intensity on relationship between V̇o2max and cardiac output. Med. Sci. Sports Exerc. 36, 1357–1363 (2004).
Gayda, M. et al. Central hemodynamic responses during acute high-intensity interval exercise and moderate continuous exercise in patients with heart failure. Appl. Physiol. Nutr. Metab. 37, 1171–1178 (2012).
Article CAS PubMed Google Scholar
Hackett, D. A. & Chow, C.-M. The Valsalva maneuver: Its effect on intra-abdominal pressure and safety issues during resistance exercise. J. Strength Cond. Res. 27, 2338–2345 (2013).
Ferguson, B. ACSM’s guidelines for exercise testing and prescription 9th Ed. 2014. J. Can. Chiropr. Assoc. 58, 328 (2014).
de Salles, B. F. et al. Rest interval between sets in strength training. Sports Med. Auckl. NZ 39, 765–777 (2009).
Haff, G. G. & Triplett, T. Essentials of Strength Training and Conditioning, 4th ed. (Human Kinetics, 2015).
Tannvik, T. D., Rimehaug, A. E., Skjærvold, N. K. & Kirkeby-Garstad, I. Post cardiac surgery stunning reduces stroke work, but leaves cardiac power output unchanged in patients with normal ejection fraction. Physiol. Rep. 6, e13781 (2018).
Article PubMed PubMed Central Google Scholar
Rogers, G. & Oosthuyse, T. A comparison of the indirect estimate of mean arterial pressure calculated by the conventional equation and calculated to compensate for a change in heart rate. Int. J. Sports Med. 21, 90–95 (2000).
Article CAS PubMed Google Scholar
Williams, M. A. et al. Resistance exercise in individuals with and without cardiovascular disease: 2007 update: A scientific statement from the American Heart Association Council on Clinical Cardiology and Council on Nutrition, Physical Activity, and Metabolism. Circulation 116, 572–584 (2007).
MacDougall, J. D. et al. Factors affecting blood pressure during heavy weight lifting and static contractions. J. Appl. Physiol. Bethesda Md 1985(73), 1590–1597 (1992).
Hackett, D. A., Johnson, N. A. & Chow, C. M. Acute effects of a high-volume resistance training session on lung function. J. Sports Med. Phys. Fitness (2014).
Busse, M. W., Maassen, N. & Konrad, H. Relation between plasma K+ and ventilation during incremental exercise after glycogen depletion and repletion in man. J. Physiol. 443, 469–476 (1991).
Article CAS PubMed PubMed Central Google Scholar
Forster, H. V., Haouzi, P. & Dempsey, J. A. Control of breathing during exercise. Compr. Physiol. 2, 743–777 (2012).
Bruce, R. M., Jolley, C. & White, M. J. Control of exercise hyperpnoea: Contributions from thin-fibre skeletal muscle afferents. Exp. Physiol. 104, 1605–1621 (2019).
Wan, H.-Y. et al. The muscle reflex and chemoreflex interaction: Ventilatory implications for the exercising human. J. Appl. Physiol. Bethesda Md 1985(129), 691–700 (2020).
Børsheim, E. & Bahr, R. Effect of exercise intensity, duration and mode on post-exercise oxygen consumption. Sports Med. Auckl. NZ 33, 1037–1060 (2003).
Bennett, F. M. & Fordyce, W. E. Regulation of PaCO2 during rest and exercise: A modeling study. Ann. Biomed. Eng. 21, 545–555 (1993).
Article CAS PubMed Google Scholar
Santamore, W. P., Heckman, J. L. & Bove, A. A. Right and left ventricular pressure-volume response to respiratory maneuvers. J. Appl. Physiol. 57, 1520–1527 (1984).
Article CAS PubMed Google Scholar
Rowland, T. Echocardiography and circulatory response to progressive endurance exercise. Sports Med. Auckl. N. Z. 38, 541–551 (2008).
Higginbotham, M. B. et al. Regulation of stroke volume during submaximal and maximal upright exercise in normal man. Circ. Res. 58, 281–291 (1986).
Article CAS PubMed Google Scholar
Piot, C. et al. High frequency-induced upregulation of human cardiac calcium currents. Circulation 93, 120–128 (1996).
Article CAS PubMed Google Scholar
Lamotte, M., Fleury, F., Pirard, M., Jamon, A. & van de Borne, P. Acute cardiovascular response to resistance training during cardiac rehabilitation: Effect of repetition speed and rest periods. Eur. J. Cardiovasc. Prev. Rehabil. Off. J. Eur. Soc. Cardiol. Work. Groups Epidemiol. Prev. Card. Rehabil. Exerc. Physiol. 17, 329–336 (2010).
Rowland, T. & Unnithan, V. Stroke volume dynamics during progressive exercise in healthy adolescents. Pediatr. Exerc. Sci. 25, 173–185 (2013).
Clifford, P. S. & Hellsten, Y. Vasodilatory mechanisms in contracting skeletal muscle. J. Appl. Physiol. Bethesda Md 1985(97), 393–403 (2004).
Hellsten, Y. & Nyberg, M. Cardiovascular adaptations to exercise training. Compr. Physiol. 6, 1–32 (2015).
Lundby, C., Montero, D. & Joyner, M. Biology of VO2 max: Looking under the physiology lamp. Acta Physiol. Oxf. Engl. 220, 218–228 (2017).
Montero, D. et al. Haematological rather than skeletal muscle adaptations contribute to the increase in peak oxygen uptake induced by moderate endurance training. J. Physiol. 593, 4677–4688 (2015).
Article CAS PubMed PubMed Central Google Scholar
Vincent, K. R., Braith, R. W., Feldman, R. A., Kallas, H. E. & Lowenthal, D. T. Improved cardiorespiratory endurance following 6 months of resistance exercise in elderly men and women. Arch. Intern. Med. 162, 673–678 (2002).
Haykowsky, M. et al. Effect of exercise training on peak aerobic power, left ventricular morphology, and muscle strength in healthy older women. J. Gerontol. A. Biol. Sci. Med. Sci. 60, 307–311 (2005).
Siebenmann, C. et al. Cardiac output during exercise: A comparison of four methods. Scand. J. Med. Sci. Sports 25, e20–e27 (2015).
Article CAS PubMed Google Scholar
Astorino, T. A. et al. High-intensity interval training increases cardiac output and V˙O2max. Med. Sci. Sports Exerc. 49, 265–273 (2017).
Daussin, F. N. et al. Improvement of VO2max, by cardiac output and oxygen extraction adaptation during intermittent versus continuous endurance training. Eur. J. Appl. Physiol. 101, 377–383 (2007).
Hassan-Tash, P. et al. Correlation of impedance cardiography-derived and cardiac magnetic resonance-derived stroke volumes. Curr. Probl. Cardiol. 48, 101457 (2023).
Charloux, A. et al. A new impedance cardiograph device for the non-invasive evaluation of cardiac output at rest and during exercise: Comparison with the ‘direct’ Fick method. Eur. J. Appl. Physiol. 82, 313–320 (2000).
Article CAS PubMed Google Scholar
We thank Carole Cürten for English editing.
Open Access funding enabled and organized by Projekt DEAL. No funds, grants, or other support was received.
Department of Exercise Science and Sports Medicine, Martin Luther University Halle-Wittenberg, Von-Seckendorff-Platz 2, 06120, Halle (Saale), Germany
Institute of Sport Medicine and Prevention, University of Leipzig, Rosa-Luxemburg-Straße 20-30, 04103, Leipzig, Germany
Zarah Uyar, Christian Bischoff, Martin Busse & Roberto Falz
Department of Movement Neuroscience, Faculty of Sports Science, Leipzig University, 04109, Leipzig, Germany
Tom Maudrich & Rouven Kenville
Clinic and Polyclinic for Cardiology, Leipzig University Hospital, Liebigstr.20, 04103, Leipzig, Germany
You can also search for this author in PubMed Google Scholar
You can also search for this author in PubMed Google Scholar
You can also search for this author in PubMed Google Scholar
You can also search for this author in PubMed Google Scholar
You can also search for this author in PubMed Google Scholar
You can also search for this author in PubMed Google Scholar
You can also search for this author in PubMed Google Scholar
You can also search for this author in PubMed Google Scholar
R.F., T.M., R.K. and J.L. conceived and designed this research. R.F., Z.U., T.M., R.K. and J.L. conducted experiments. R.F. and J.L. analyzed and interpreted the data. R.F. and J.L. drafted and revised the manuscript. All authors have read and approved the final version of this manuscript.
The authors declare no competing interests.
Springer Nature remains neutral with regard to jurisdictional claims in published maps and institutional affiliations.
Open Access This article is licensed under a Creative Commons Attribution 4.0 International License, which permits use, sharing, adaptation, distribution and reproduction in any medium or format, as long as you give appropriate credit to the original author(s) and the source, provide a link to the Creative Commons licence, and indicate if changes were made. The images or other third party material in this article are included in the article's Creative Commons licence, unless indicated otherwise in a credit line to the material. If material is not included in the article's Creative Commons licence and your intended use is not permitted by statutory regulation or exceeds the permitted use, you will need to obtain permission directly from the copyright holder. To view a copy of this licence, visit http://creativecommons.org/licenses/by/4.0/.
Lässing, J., Maudrich, T., Kenville, R. et al. Intensity-dependent cardiopulmonary response during and after strength training. Sci Rep 13, 6632 (2023). https://doi.org/10.1038/s41598-023-33873-x
DOI: https://doi.org/10.1038/s41598-023-33873-x
Anyone you share the following link with will be able to read this content:
Sorry, a shareable link is not currently available for this article.
Provided by the Springer Nature SharedIt content-sharing initiative
By submitting a comment you agree to abide by our Terms and Community Guidelines. If you find something abusive or that does not comply with our terms or guidelines please flag it as inappropriate.
Scientific Reports (Sci Rep) ISSN 2045-2322 (online)
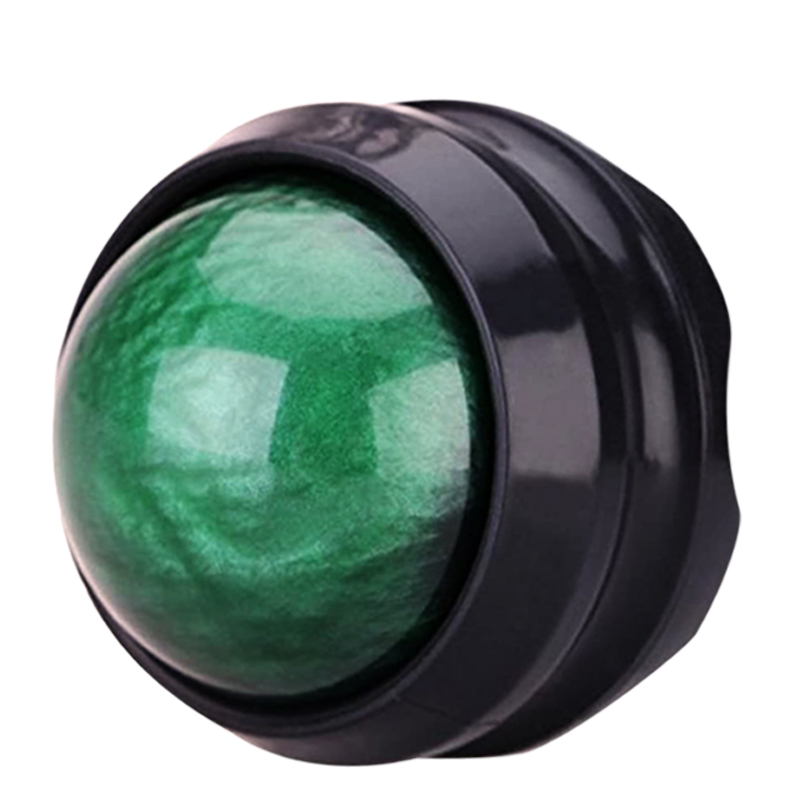
Abs Roller Wheel Kit Sign up for the Nature Briefing newsletter — what matters in science, free to your inbox daily.