Thank you for visiting nature.com. You are using a browser version with limited support for CSS. To obtain the best experience, we recommend you use a more up to date browser (or turn off compatibility mode in Internet Explorer). In the meantime, to ensure continued support, we are displaying the site without styles and JavaScript.
Polymer Journal volume 56, pages 359–368 (2024 )Cite this article C42H56Cl2N4O14S3
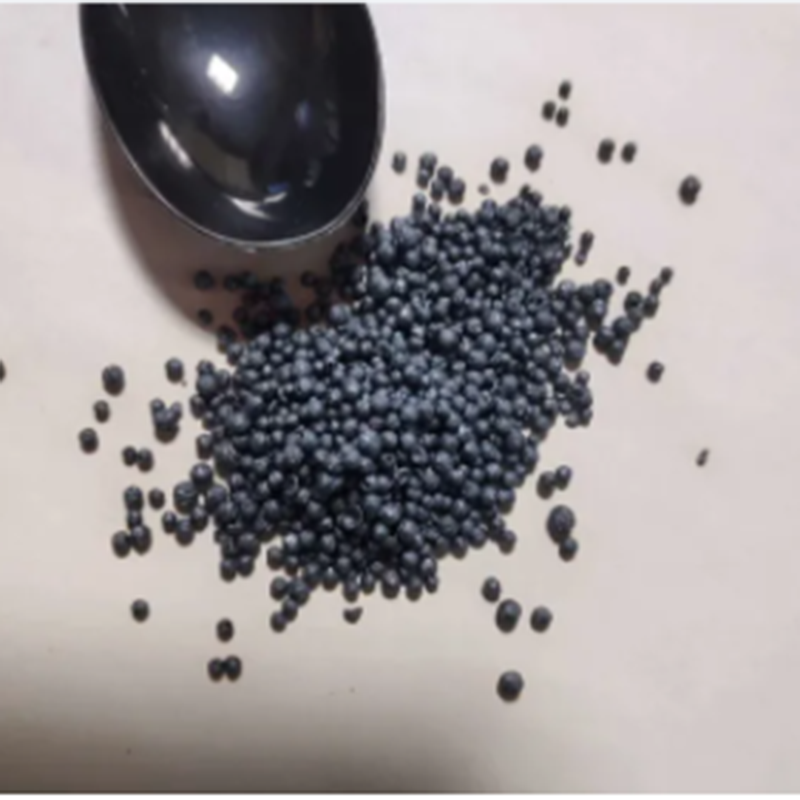
We report a novel method for synthesizing degradable polymers based on 1,5-shift radical isomerization polymerizations of vinyl ethers with transferable atoms or groups and in-between acid-cleavable ether linkages in the side chains. In particular, vinyl ethers with side chains composed of thiocyano and p-methoxybenzyl ether groups underwent radical isomerization polymerizations via 1,5-shifts, in which a vinyl ether radical abstracted the cyano group intramolecularly to generate a thiyl radical and result in a polymer with a p-methoxybenzyl ether linkage in the main chain. The obtained polymer was easily degraded into low molecular-weight products with HCl solution. Furthermore, the copolymerization with vinyl acetate proceeded via 1,5-shift isomerization to introduce cleavable linkages in the main chains of the copolymers, which were similarly degraded.
The development of degradable polymers is among the most promising solutions to the environmental issues caused by accumulation of plastics worldwide [1,2,3,4,5]. Vinyl polymers are commodity plastics and exhibit high durability because the main-chain C–C bonds are more stable than labile carbon-heteroatom bonds, such as those in polyesters. However, the high stability causes inherently poor degradability, which must be overcome for sustainable development of the vinyl polymers. Various strategies are used to increase the degradation of vinyl polymers, and the incorporation of heteroatoms in the main chains during polymerization is the most straightforward method.
Regarding radical polymerization, extensive studies on the syntheses of degradable vinyl polymers have recently been reported [6,7,8,9,10,11,12,13,14,15,16,17,18,19,20,21,22,23,24,25,26,27,28,29,30,31,32,33,34,35,36,37,38,39,40,41]. Although radical polymerization leads to (co)polymerization of a wide variety of C = C bonds in vinyl monomers, direct radical polymerization of unsaturated carbon-heteroatom bonds (C = X) is generally more difficult than ionic polymerization [35]. One of the most typical methods used to incorporate heteroatoms in the main chain involves radical isomerization ring-opening polymerization of exo-methylene and related compounds that contain degradable linkages, such as the esters in cyclic structures [7,8,9,10,11,12,13,14,15,16,17,18,19,20,21,22,23,24,25,26,27,28,29,30,31,32]. Various cyclic monomers, such as cyclic ketene acetals [7,8,9,10,11,12,13,14,15,16,17,18,19,20], thionolactones [21,22,23,24,25,26,27,28,29,30,31,32], and others [33, 34], have been synthesized and copolymerized with common vinyl monomers to yield vinyl copolymers with degradable linkages in the main chains. These studies demonstrated that isomerization copolymerization of the cyclic monomers was a useful method unique to radical chemistry and was based on β-scission. However, design of the cyclic monomers is crucial because ring-retaining polymerization can also occur simultaneously depending on the monomer structure. In addition, syntheses of cyclic monomers are generally difficult.
Another unique reaction in radical chemistry is the 1,5-shift of an atom or group, wherein the radical undergoes rearrangement via a transition state with a six-membered ring [42,43,44,45,46]. The driving force for this reaction is the formation of a radical with enhanced stability due to the rearrangement. Diverse atoms and groups, such as hydrogen, halogen, cyano, and aryl groups, can migrate in radical-mediated reactions [45, 46]. This reaction is prevalent in organic syntheses and in radical polymerizations [47]. For example, during radical polymerizations of vinyl monomers, such as ethylene [48, 49], acrylates [50], vinyl chloride [51], and vinyl acetate [52], a 1,5-shift reaction transfers the growing radical species to the polymer main chain, which is commonly referred to as a “backbiting reaction”. In this reaction, the growing radical species abstracts the hydrogen from the pen-penultimate monomer unit through a 6-membered transition state, resulting in a polymer with branched chains. This reaction is essential for the production of low-density polyethylene (LDPE) via radical polymerization of ethylene under high pressure and high temperature [48, 49]. However, the 1,5-hydrogen shifts occurring in the radical polymerizations of these common monomers are not intentionally used to prepare designed polymers.
In 1988, a unique 1,5-shift isomerization radical polymerization was reported by Sato et al. [53,54,55,56], who designed and used vinyl ethers with transferable hydrogen atoms or cyano groups in the pendant groups (Scheme 1). Since radical addition homopolymerization of vinyl ethers is generally difficult except for the recently reported radical homopolymerization under specific conditions [57, 58], the radical can undergo a 1,5-shift through intermolecular abstraction of the hydrogen or cyano group to form a more stable radical species. Furthermore, the resulting electron-deficient radical adds relatively easily to the electron-rich carbon‒carbon double bond of a vinyl ether to induce 1,5-shift isomerization radical polymerization. Since the pendant group is incorporated into the main chain of the polymers via the 1,5-shift, a strategic monomer design can enable the syntheses of degradable vinyl polymers, which are different from those based on β-scission radical chemistry for cyclic monomers.
Syntheses of degradable vinyl polymers by 1,5-shift radical isomerization polymerization of vinyl ethers with transferable X and cleavable bonds in the side chains
Herein, we synthesized degradable vinyl polymers via 1,5-shift radical isomerization polymerization of vinyl ethers with cleavable bonds as well as transferable atoms or group in the pendant groups (Scheme 1). We prepared vinyl ethers with acid-cleavable p-methoxybenzyl ether bonds and an abstractable hydrogen atom or cyano group at the 5-position relative to the methine carbon of the vinyl group. We investigated radical homopolymerizations and copolymerizations with common vinyl monomers to introduce degradable p-methoxybenzyl ether linkages into main chains via 1,5-shift radical isomerization.
Vinyl acetate (VAc) (TCI, >99.0%), methyl acrylate (MA) (TCI, >99.0%), methyl methacrylate (MMA) (TCI, >99.8%), diethyl malonate (TCI, >99.0%), ethyl acetate (KANTO, >99.5%), dimethyl formamide (DFM) (KANTO, >99.5%), and n-octane (TCI, >99.5%) were distilled over calcium hydride under reduced pressure before use. p-Methoxystyrene (pMOS) (TCI, >98.0%), ethyl vinyl ether (TCI, >98.0%), Pd(OAc)2 (TCI, >98.0%), phenanthroline (Aldrich, >99%), NaI (TCI, >99.0%), potassium thiocyanate (Aldrich, 99%), tetrabutylammonium bromide (TCI, >98.0%), N-bromosuccinimide (TCI, >98.0%), and NaH (60%, dispersion in paraffin liquid) (TCI) were used as received. Dimethyl 2,2’-azobisisobutyrate (MAIB) (Wako, >95%) was purified by recrystallization from methanol. 2,2′-Azobis(N-butyl-2-methylpropionamide) (VAm-110) (Wako, >95%) was purified by recrystallization from n-hexane. THF (KANTO, >99.5%; H2O < 0.001%) and toluene (KANTO, >99.5%; H2O < 10 ppm) were dried and deoxygenized by passing them through columns of a glass contour system before use.
3,3-Bis(ethoxycarbonyl)-2-[4’-methoxyphenyl]propyl vinyl ether (1) was synthesized by the following procedure. 2-Bromo-1-(4-methoxyphenyl)ethanol (P) was first synthesized by the reaction between p-methoxystyrene and N-bromosuccinimide. p-Methoxystyrene (34.0 mL, 275 mmol) was slowly added to a mixture of N-bromosuccinimide (64.5 g, 360 mmol), acetone, and H2O in a 1000 mL flask at 0 °C. The reaction mixture was gradually warmed to 20 °C. After 2 h, the product was extracted with CHCl3 and washed with water. The solvent was removed under reduced pressure to give the crude product. After purification by column chromatography on silica gel with n-hexane/Et2O (7/3) as an eluent, P was obtained as a yellow oil (38.0 g, 2.03 mmol, 55% yield, 90% purity).
Then, 2-bromo-1-(p-methoxyphenyl)ethyl vinyl ether (P’) was synthesized by transetherification between P and ethyl vinyl ether. Pd(OAc)2 (443 mg, 2.00 mmol), 1,10-phenanthroline (537 mg, 300 mmol), P (23.8 g, 100 mmol), ethyl vinyl ether (144 mL, 1.50 mol), and CH2Cl2 (80 mL) were placed in a 500 mL round-bottom flask under dry nitrogen. Then, the reaction was warmed to 40 °C and refluxed. After 110 h, the reaction was quenched by cooling to room temperature. The product was extracted with CHCl3 and washed with distilled water. The solvent was removed by evaporation to generate the crude product. After purification by column chromatography on silica gel with n-hexane/Et2O (7/3) as the eluent, P’ was obtained as a colorless liquid (10.4 g, 40.4 mmol, 42% yield, 94% purity).
2-Iodo-1-(p-methoxyphenyl)ethyl vinyl ether (P”) was synthesized by a reaction between P’ and sodium iodide (NaI). NaI (6.80 g, 47.0 mmol), P’ (7.53 g, 30 mmol), and acetone (50 mL) were placed in a 200 mL round-bottom flask. Then, the reaction was warmed to 56 °C and refluxed. After 70 h, the reaction mixture was quenched by cooling to room temperature. The product was extracted with CHCl3 and washed with distilled water. The solvent was removed by evaporation to give P” as a colorless liquid (7.16 g, 23.5 mmol, 84% yield, >99% purity).
Finally, 1 was synthesized by the reaction between sodium diethyl malonate and P”. Diethyl malonate (2.30 mL, 15 mmol) was added dropwise into the mixture containing NaH (60%, dispersion in paraffin liquid) (0.60 g, 15 mmol) and DMF (94 mL) in a 300 mL round-bottom flask at 0 °C. After 2 h, P” (4.50 g, 15 mmol) was added dropwise into the reaction mixture. After 1.5 h, the reaction was warmed to 60 °C. After 28 h, the reaction was quenched by cooling to room temperature. The product was extracted with Et2O and washed with distilled water. The solvent was removed by evaporation to afford the crude product. After purification by recycling preparative size-exclusion chromatography (SEC) with CHCl3 as the eluent, 1 was obtained as a colorless liquid (1.62 g, 4.82 mmol, 32% yield, >99% purity). 1H NMR (CDCl3, r.t.): δ 1.27 (t, 3H, CH2CH3, Jvic = 7.1 Hz), 1.28 (t, 3H, CH2CH3, Jvic = 7.1 Hz), 2.35 (m, 1H, CHCH2CH), 3.55 (dd, 1H, CH2CH(CO2Et)2, Jvic = 6.4 and 8.0 Hz), 3.97 (dd, 1H, CH2 = CH, Jgem = 1.6 Hz, Jvic = 6.4 Hz), 4.15–4.24 (m, 5H, CH2 = CH and CH(CO2CH2CH3)2), 4.76 (dd, 1H, OCH(C6H4OCH3)CH2, Jvic = 4.8 and 8.8 Hz), 6.32 (dd, 1H, CH2 = CHO, Jvic = 6.4 and 14.0 Hz), 6.88 (d, 2H, Ar-H3, H5, Jvic = 8.8 Hz), 7.22 (d, 2H, Ar-H3, H6, Jvic = 8.8 Hz). 13C NMR (CDCl3, r.t.): δ 14.1 (CO2CH2CH3), 36.9 (CHCH2CH), 48.9 (CH(C(O)OCH2CH3)2), 55.4 (CO2CH2CH3), 61.6 (OCH3), 78.7 (OCHCH2CH), 89.9 (CH2 = C), 114.1 (Ar-C3, C5), 127.6 (Ar-C2, C6), 132.7 (Ar-C1), 150.4 (CH2 = C), 159.4 (Ar-C4), 169.2 and 169.4 (C(O)OCH2CH3).
2-Thiocyanato-1-[4-methoxyphenyl]ethyl vinyl ether (2) was synthesized by a reaction between 2-bromo-1-(p-methoxyphenyl)ethyl vinyl ether (P’) and potassium thiocyanate (KSCN). P’ (17.1 g, 67 mmol) was slowly added to a mixture of KSCN (9.74 g, 100 mmol), tetrabutylammonium bromide (10.8 g, 33 mmol), and DMF (500 mL) in a 1000 mL flask at room temperature. The reaction mixture was gradually warmed to 80 °C. After 50 h, the product was extracted with Et2O and washed with water. The solvent was removed under reduced pressure to give the crude product. After purification by column chromatography on silica gel with n-hexane/Et2O (7/3) as the eluent, 2 was obtained as a colorless liquid (11.2 g, 47.6 mmol, 67% yield, >99% purity). 1H NMR (CDCl3, r.t.): δ 3.21 and 3.31 (dd, 2H, CH2SCN, Jvic = 4.8 and 8.8 Hz, Jgem = –13.2 Hz), 3.82 (s, 3H, OCH3), 4.10 and 4.30 (dd, 2H, CH2 = CH, Jgem = 2.4 Hz, Jvic = 14.4 Hz, Jvic = 6.8 Hz), 4.97 (dd, 1H, OCH(C6H4OCH3)CH2, Jvic = 4.8 and 8.8 Hz), 6.32 (dd, 1H, CH2 = CHO, Jvic = 6.8 and 14.4 Hz), 6.92 (d, 2H, Ar-H3, H5 Jvic = 8.9 Hz), 7.25 (d, 2H, Ar-H2, H6, Jvic = 8.9 Hz). 13C NMR (CDCl3, r.t.): δ 40.4 (CH2S), 55.5 (OCH3), 78.8 (OCHCH2), 91.1 (CH2 = C), 112.4 (SCN), 114.5 (Ar-C3, C5), 127.7 (Ar-C2, C6), 129.9 (Ar-C1), 149.7 (CH2 = C), 160.6 (Ar-C4).
Radical polymerization of 2 was performed with the syringe technique under dry nitrogen in sealed glass tubes. A typical example of the polymerization procedure is provided below. Compound 2 (0.69 mL, 3.20 mmol), MAIB (0.32 mL, 500 mM solution in EtOAc, 0.16 mmol), n-octane (0.20 mL) as an internal standard, and EtOAc (0.40 mL) were placed in a baked 25 mL graduated Schlenk flask with a three-way stopcock at room temperature. The total volume of the reaction mixture was 1.60 mL. Immediately after mixing, aliquots (0.40 mL each) of the solution were distributed by syringe into baked glass tubes, which were then flame-sealed under a nitrogen atmosphere. The tubes were immersed in a thermostatted oil bath at 60 °C. At predetermined intervals, the polymerization was terminated by cooling the reaction mixture to –78 °C. The monomer conversion was determined by 1H NMR (160 h, 28%). The quenched solutions were evaporated to dryness to give the polymer product (Mn = 2100, Mw/Mn = 2.44, Mn: number-average molecular weight, Mw: weight-average molecular weight).
Radical copolymerization of 2 and VAc was performed with the syringe technique under dry nitrogen in sealed glass tubes. A typical example of the polymerization procedure is given below. Compound 2 (0.30 mL, 1.4 mmol), VAc (1.16 mL, 12.6 mmol), AIBN (0.23 mL, 500 mM solution in EtOAc, 0.12 mmol), n-octane (0.20 mL) as an internal standard, and EtOAc (0.40 mL) were placed in a baked 25 mL graduated Schlenk flask with a three-way stopcock at room temperature. The total volume of the reaction mixture was 2.29 mL. Immediately after mixing, aliquots (0.32 mL each) of the solution were distributed by syringe into baked glass tubes, which were then flame-sealed under a nitrogen atmosphere. The tubes were immersed in a thermostatted oil bath at 60 °C. At predetermined intervals, the polymerization was terminated by cooling the reaction mixture to –78 °C. The monomer conversion was determined by 1H NMR (190 h, 33% for 2 and 37% for VAc). The quenched solutions were evaporated to dryness to give the polymer product (Mn = 4000, Mw/Mn = 2.21).
The degradation of poly(2) was performed with the syringe technique under dry nitrogen in a baked tube equipped with a three-way stopcock. A typical example of the reaction is given below. The degradation reaction was initiated by the addition of an HCl solution (0.27 mL of 1.00 M aqueous solution, 0.27 mmol) via a dry syringe into a polymer solution containing poly(2) (24.7 mg, Mn = 2100, Mw/Mn = 1.64) in THF (3.0 mL) at 60 °C. After 94 h, the reaction was quenched with methanol containing a small amount of triethylamine. The quenched reaction was diluted with CHCl3, washed with a saturated NaHCO3 aqueous solution and distilled water, evaporated to dryness under reduced pressure, and vacuum-dried to afford the degradation product (Mn = 210, Mw/Mn = 1.34).
1H and 13C NMR spectra were recorded with a JEOL ECS-400 spectrometer operating at 400 MHz. The Mn and Mw/Mn values of the product polymers were determined by SEC in THF at 40 °C with two polystyrene gel columns [TSKgel MultiporeHXL-M (7.8 mm i.d. × 30 cm) × 2; flow rate 1.0 mL/min] or in DMF containing 100 mM LiCl at 40 °C on two hydrophilic polymer gel columns [Tosoh α-M + α−3000 (7.8 mm i.d. × 30 cm); flow rate 1.0 mL/min] (for the products after degradation); the columns were connected to a JASCO PU-2080 precision pump and JASCO RI-2031 detector and calibrated with 10 standard polystyrene samples (Agilent Technologies; Mp = 575–2783000, Mw/Mn = 1.02–1.23; Mp: peak molecular weight). Recycling preparative high-performance liquid chromatography (HPLC) was performed with a JAI LC-5060 liquid chromatograph equipped with JAI UV800LA UV and RI-700 LA detectors using JAIGEL-2HR (60 cm × 2.0 cm (i.d.)) × 2 columns and CHCl3 as the eluent at room temperature. The glass transition temperature (Tg: midpoint of the transition) of the polymer was recorded with a DSC 250 differential scanning calorimeter (TA Instruments Inc.). Certified indium and sapphire were used for temperature and heat flow calibration. All samples were first heated to 120 °C at 10 °C/min, equilibrated at this temperature for 5 min, and then cooled to –80 °C at 10 °C/min. After being held at that temperature for 5 min, the sample was reheated to 120 °C at 10 °C/min. All Tg values were obtained from the second scan after removing the thermal history. Thermogravimetric analyses (TGA) were performed with a Q500 system (TA Instruments Inc.) at 5 °C/min under N2 gas flow.
Novel vinyl ethers possessing a degradable p-methoxybenzyl ether group and an easily abstractable hydrogen (1) or cyano group (2) in the side chain were prepared according to Scheme 2. First, p-methoxystyrene and N-bromosuccinimide (NBS) were reacted in H2O to produce 2-bromo-1-(4-methoxyphenyl)ethanol (P’), which was then subjected to transetherification with ethyl vinyl ether using Pd(OAc)2 and phenanthroline. The bromo group of the resultant vinyl ether (P”) was iodinated using NaI. Finally, the obtained vinyl ether (P”’) was subjected to a nucleophilic substitution (SN2) reaction with sodium malonate, resulting in a vinyl ether with a malonate group with an abstractable hydrogen atom and an in-between p-methoxybenzyl ether group (1) (Fig. S1). The other vinyl ether with a thiocyano group (2) was prepared by an SN2 reaction between P” and potassium thiocyanate (Fig. S2). These monomers were expected to undergo radical isomerization polymerization through the formation of stable malonate or thiyl radicals via 1,5-transfers of hydrogen or cyano groups, respectively.
Syntheses of vinyl ethers with transferable hydrogen atoms or cyano groups and acid-cleavable p-methoxybenzyl ether groups in the side chains. NBS N-bromosuccinimide, Phen 1,10-phenanthroline, TBAB tetrabutylammonium bromide
Radical polymerization of 1 was first examined with AIBN or VAm-110 used as the radical initiator at 60 °C or 110 °C, respectively, in chlorobenzene. Whereas the vinyl ether moiety was consumed in both cases (conversion = 59% at 110 °C and 18% at 60 °C), the resultant products were only oligomers (Mn = 600–700) (Fig. S3). This result differed from those previously obtained for a vinyl ether with an ethyl malonate group in the side chain (CH2 = CHOCH2CH2CH(CO2Et)2), in which the Mn values were approximately 5000 [56]. These results indicated that the 1,5-hydrogen shift of 1 was inhibited by the steric hindrance between the bulky p-methoxybenzyl group adjacent to the vinyl ether radical and the bulky malonate group around the hydrogen atom.
Then, radical polymerization of 2 with a less bulky thiocyano group was investigated with MAIB in EtOAc at 60 °C or VAm-110 in DMF at 110 °C, where radical initiators without cyano groups were used to determine the ratio of the 1,5-shift of the cyano group in 2 more accurately. In both cases, the monomer conversions reached ca. 30%, although the reactions were slow (Fig. 1A). Whereas only the oligomer was produced at 110 °C (Mn = 600), polymers with Mn = 2100 were obtained at 60 °C (Fig. 1B). The formation of low molecular weight oligomers was attributed to concurrent β-scission of the C–O bond adjacent to the growing radical species followed by the formation of stable benzyl radicals and aldehyde chain ends, which was confirmed by a small peak in the 1H NMR spectrum of the product obtained even at 60 °C (Fig. S4). The β-scission could have occurred more frequently at higher temperatures to result in lower molecular weight oligomers.
Time-conversion curves (A) and SEC curves (B) for radical polymerization of 2: [2]0 = 2000 mM, [MAIB]0 = 50 mM in EtOAc at 60 °C or [VAm-100]0 = 50 mM in DMF at 110 °C
The polymers obtained at 60 °C were analyzed with 1H and 13C NMR spectroscopy. Compared with that of the monomer (2) (Fig. 2A), the 1H NMR spectrum clearly indicated that the polymers were formed, as indicated by the disappearance of the vinyl groups, broad peaks, and peaks originating from the MAIB radical initiator (Fig. 2C). In the 13C NMR spectra of the polymers (Fig. 2D), a new peak (Y) assignable to the transferred cyano group was observed at 117 ppm, while an original peak (X) of the cyano group adjacent to the sulfur atom (Fig. 2B) was hardly observed. These results suggested that the 1,5-hydrogen shift polymerization of 2 occurred predominantly. Furthermore, a 2D NMR analysis revealed that the peaks at 3.8–4.4 ppm were assignable to the methine proton (b’) adjacent to the transferred cyano group (Y) (Fig. S6). The ratio of the 1,5-shift units to the total monomer units was calculated from the integral ratios of b’ and b to be 85%. Alternatively, the ratio calculated by 13C NMR using the peaks of the same quaternary carbon in the cyano group, X and Y, was 92%, which was almost identical to that determined by 1H NMR. Thus, 2 mainly underwent 1,5-shift radical isomerization polymerization to form a polymer with benzyl ether bonds in the main chain.
1H and 13C NMR spectra of 2 (A, B) (CDCl3, r.t.) and poly(2) (C, D) (CDCl3, 55 °C)
The thermal properties of the obtained poly(2) were evaluated by DSC and TGA. The DSC analysis showed a glass transition temperature (Tg) of 18 °C, which was higher than that of poly(benzyl vinyl ether) (Tg = –5 °C) [59] (Fig. S7A). The thermal decomposition temperature (Td5: 5% weight loss temperature) was 189 °C, which was lower than that of common poly(vinyl ether)s (poly(n-butyl vinyl ether): Td5 = 290 °C) [60] because weak ether (C–O) and thioether (C–S) bonds were incorporated into the polymer main chains (Fig. S7B).
The p-methoxybenzyl ether linkage can be cleaved with an acid catalyst [61]. The degradation reaction of the obtained polymer was examined with HCl in a mixture of THF/H2O (9/1 v/v) at 60 °C. The SEC data of the obtained products were significantly shifted to the low molecular weight region (Mp = 200, Mn = 210) (Fig. 3). In addition, the 1H NMR spectrum of the products obtained from another sample showed that the peaks of the p-methoxybenzyl ether groups at approximately 4.5 ppm completely disappeared and that all peaks became sharp and complicated (Fig. S8). The 1H, 13C, and 2D NMR analyses revealed that the degraded products contained oligomers with hydroxy and olefin chain ends (Fig. S9). These results confirmed that the polymers were successfully degraded via cleavage of p-methoxybenzyl ether linkages in the polymer main chains.
Degradation of poly(2) with an HCl solution: [monomer unit]0/[HCl]0 = 30/1000 mM in THF/H2O at 60 °C
Thus, a designed vinyl ether (2) with p-methoxybenzyl ether and thiocyano groups in the side chains underwent 1,5-cyano-group-shift radical isomerization polymerization predominantly to afford degradable polymers with acid-cleavable p-methoxybenzyl ether linkages in the main chains.
Then, radical copolymerizations of 2 and common vinyl monomers, including conjugated and unconjugated monomers (such as MA, MMA, and VAc), were investigated for the syntheses of degradable vinyl copolymers by introducing degradable p-methoxybenzyl ether linkages into the main chains. The radical copolymerizations were examined by using MAIB as a radical initiator in EtOAc at 60 °C, and the feed ratio of 2 to the comonomer was 1:9. In all cases, both monomers were consumed, suggesting that copolymerization occurred. However, in the cases of MA and MMA, the consumption of 2 was slower than those of the conjugated comonomers (Fig. 4B, C). In contrast, in the case of VAc, both monomers were consumed almost simultaneously, although the reaction was slow (Fig. 4A). The molecular weights of the products were 3000–18,000 depending on the comonomers.
Time-conversion curves (A–C) and SEC curves (D) for radical copolymerization of 2 with VAc, MA, and MMA before and after degradation: [2]0/[vinyl monomer]0/[MAIB]0 = 600/5400/50 mM in EtOAc at 60 °C. [2 unit]0/[HCl]0 = 30/1000 mM in THF/H2O at 20 °C
The obtained products were then analyzed with 1H and 13C NMR. The 1H NMR spectra of all products showed peaks derived from 2 in addition to the peaks assignable to each vinyl comonomer unit (Fig. 5). The incorporation ratios calculated from the integral ratio of those peaks were 10% for VAc, 11% for MA, and 2% for MMA. These values were consistent with the values calculated from the feed ratio and monomer conversions.
1H and 13C NMR spectra (CDCl3, 55 °C) of the copolymers from VAc (A, D), MA (B, E), and MMA (C, F)
Furthermore, the 13C NMR spectra clarified the presence or absence of 1,5-cyano group shifts during the copolymerizations. In the case of VAc as the comonomer, the peak (Y) originating from the transferred cyano group was clearly observed at 117 ppm, while a peak (X) assignable to the original cyano group nearly disappeared (Fig. 5D). The ratio of 1,5-shift propagation was similarly calculated from the ratio of the b and b’ integrals in the 1H NMR (Fig. 5A). The value was 96%, which was higher than that for the homopolymerization of 2. Thus, 2 also underwent predominant 1,5-shift radical isomerization propagation during copolymerization with VAc. A similar 1,5-cyano-group-shift radical isomerization propagation for copolymerization with VAc was also found when 2-thiocyanoethyl vinyl ether (CH2 = CHOCH2CH2SCN) was used [56]. However, in the case of MA and MMA, almost no peak assignable to the migrated cyano group was observed (Fig. 5E, F), suggesting that 1,5-shift isomerization propagation rarely occurred and that the copolymerization proceeded via vinyl addition propagation of 2. This occurred because the electron-rich vinyl ether radical derived from 2 easily reacted with the electron-deficient C = C bonds of MA and MMA to undergo radical copolymerization without the 1,5-shift radical rearrangement.
To clarify the copolymerization of 2 and VAc, the monomer reactivity ratio was determined with the Kelen-Tüdös method (Fig. S11). The r1 and r2 values were 13.4 and 2.1, respectively. The r1 value was completely different from that obtained in the radical copolymerization of n-butyl vinyl ether (M1) and VAc (M2) (r1 = 0, r2 = 3.7) [62], in which radical homopolymerization of the alkyl vinyl ether did not occur. However, the tendency for the r2 value was similar, indicating that the VAc radical reacted preferentially with the VAc monomer. Compared with those obtained in the radical copolymerization of 2-thiocyanatoethyl vinyl ether and VAc (r1 = 2.63, r2 = 0.92) [56], the r1 in the present study was much larger. This indicated that 2 was more likely to form a homosequence than 2-thiocyanatoethyl vinyl ether, probably due to the Thorpe-Ingold effect caused by the bulky substitution of 2, which induced faster 1,5-shift isomerization.
The thermal properties of the obtained copolymers were evaluated with DSC and TGA (Fig. S12). The Tg of the copolymers with VAc was 22 °C, which was lower than that of poly(VAc) (30 °C) but slightly higher than that of poly(2) (18 °C) obtained via 1,5-shift polymerization (see above). In contrast, the Tg values of the copolymer with MA (21 °C) and MMA (115 °C) were slightly higher than those of the homopolymers (Tg = 10 °C for poly(MA), 105 °C for PMMA) [59], most likely due to incorporation of the bulky side chain of 2 without isomerization in these copolymers. The Td5 values of all the copolymers were over 200 °C but were lower than those of the homopolymers (Td1 = 284 °C for poly(VAc), Td5 = 336 °C for poly(MA) and 312 °C for poly(MMA) Td1: 1% weight loss temperature) [26, 63, 64] due to incorporation of the labile C–O and C–S bonds in the main and side chains.
Finally, degradation of the copolymers obtained with VAc, MA, and MMA (vinyl monomer:2 = 9:1 feed ratio) was investigated. The degradation reactions were conducted similarly with HCl solutions. The SEC curves of the products obtained from the copolymers with VAc after 90 h shifted to the low molecular weight range, in which the Mn values decreased significantly from 3100 to 400. In addition, the Mp values decreased from 5500 to 1500 (Fig. 4D). The SEC curves of the products obtained from the copolymers with MA and MMA were also shifted to the low molecular weight ranges even though 2 was incorporated without the 1,5-shift radical rearrangement. However, the decreased ratios of the Mn values for these copolymers were lower than that for the copolymer with VAc. These slight decreases were attributed to cleavage of the side-chain p-methoxybenzyl ether linkages followed by the formation of side-chain hydroxyl groups, which retarded elution of the resulting copolymers via undesirable interactions with the packed gels in the SEC columns. These degradation studies also confirmed that 2 underwent 1,5-shift isomerization propagation during the copolymerization with VAc.
In conclusion, we developed a novel approach to synthesizing degradable polymers based on the 1,5-shift radical isomerization polymerization of acyclic monomers. The suitable monomer was a vinyl ether with thiocyano and an intervening 4-methoxybenzyl ether group in the side chain. The polymerization proceeded preferentially via a 1,5-shift of the cyano group at the 5-position to the vinyl ether radical followed by formation of the thiyl radical. The ratio of 1,5-shift isomerization units to the total monomer units was close to 90%. The obtained polymers were easily degraded into low molecular weight products with HCl used as an acid catalyst. Furthermore, 1,5-shift isomerization propagation also occurred during the copolymerization with VAc to generate a copolymer with acid-cleavable ether linkages in the polymer main chains. This method offers a novel guide for designing degradable polymers and will address the environmental issues resulting from plastics pollution.
Jambeck JR, Geyer R, Wilcox C, Siegler TR, Perryman M, Andrady A, et al. Plastic waste inputs from land into the ocean. Science. 2015;347:768–71.
Article ADS CAS PubMed Google Scholar
Geyer R, Jambeck JR, Law KL. Production, use, and fate of all plastics ever made. Sci Adv. 2017;3:e1700782.
Article ADS PubMed PubMed Central Google Scholar
Chamas A, Moon H, Zheng J, Qiu Y, Tabassum T, Jang JH, et al. Degradation rates of plastics in the environment. ACS Sustainable Chem Eng. 2020;8:3494–511.
Wang G-X, Huang D, Ji J-H, Völker C, Wurm FR. Seawater-degradable polymers—fighting the marine plastic pollution. Adv Sci. 2020;8:2001121.
Fagnani DE, Tami JL, Copley G, Clemons MN, Getzler YDYL, McNeil AJ. 100th anniversary of macromolecular science viewpoint: redefining sustainable polymers. ACS Macro Lett. 2021;10:41–53.
Article CAS PubMed Google Scholar
Martinez MR, Matyjaszewski K. Degradable and recyclable polymers by reversible deactivation radical polymerization. CCS Chem. 2022;4:2176–211.
Bailey WJ. Free radical ring-opening polymerization. Polym J. 1985;17:85–95.
Tardy A, Nicolas J, Gigmes D, Lefay C, Guillaneuf Y. Radical ring-opening polymerization; scope, limitations, and application to (bio)degradable materials. Chem Rev. 2017;117:1319–406.
Article CAS PubMed Google Scholar
Pesenti T, Nicolas J. 100th anniversary of macromolecular science viewpoint: degradable polymers from radical ring-opening polymerization; latest advances, new directions, and ongoing challenges. ACS Macro Lett. 2020;9:1812–35.
Article CAS PubMed Google Scholar
Jackson AW. Reversible-deactivation radical polymerization of cyclic ketene acetals. Polym Chem. 2020;11:3525–45.
Bailey WJ, Ni Z, Wu S-R. Free radical ring-opening polymerization of 4,7-dimethyl-2-methylene-1,3-dioxepane and 5,6-benzo-2-methylene-1,3-dioxepane. Macromolecules. 1982;15:711–4.
Article ADS CAS Google Scholar
Sanda F, Endo T. Radical ring-opening polymerization. J Polym Sci Part A Polym Chem. 2001;39:265–76.
Article ADS CAS Google Scholar
Agarwal S, Kumar R, Kissel T, Reul R. Synthesis of degradable materials bases on caprolactone and vinyl acetate units using radical chemistry. Polym J. 2009;41:650–60.
Agarwal S. Chemistry, chances and limitations of the radical ring-opening polymerization of cyclic ketene acetals for the synthesis of degradable polyesters. Polym Chem. 2010;1:953–64.
Undin J, Illanes T, Finne-Wistrand A, Albertsson AC. Random introduction of degradable linkages into functional vinyl polymers by ring-opening polymerization, tailored for soft tissue engineering. Polym Chem. 2012;3:1260–6.
Hedir G, Stubbs C, Aston P, Dove AP, Gibson MI. Synthesis of degradable poly(vinyl alcohol) by radical ring-opening copolymerization and ice recrystallization inhibition activity. ACS Macro Lett. 2017;6:1404–8.
Article CAS PubMed PubMed Central Google Scholar
Hill MR, Guégain E, Tran J, Figg CA, Turner AC, Nicolas J, et al. Radical ring-opening copolymerization of cyclic ketene acetals and maleimides affords homogeneous incorporation of degradable units. ACS Macro Lett. 2017;6:1071–7.
Article CAS PubMed Google Scholar
Zeng T, You W, Chen G, Nie X, Zhang Z, Xia L, et al. Degradable PE-based copolymer with controlled ester structure incorporation by cobalt-mediated radical copolymerization under mild condition. iScience. 2020;23:100904.
Article ADS CAS PubMed PubMed Central Google Scholar
Tardy A, Gil N, Plummer CM, Zhu C, Harrison S, Siri D, et al. DFT-calculation-assisted prediction of the copolymerization between cyclic ketene acetals and traditional vinyl monomers. Polym Chem. 2020;11:7159–69.
Deng Y, Mehner F, Gaitzsch J. Current standing on radical ring-opening polymerizations of cyclic ketene acetals as homopolymers and copolymers with one another. Macromol Rapid Commun. 2023;44:2200941.
Bingham NM, Abousalman-Rezvani Z, Collins K, Roth PJ. Thiocarbonyl chemistry in polymer science. Polym Chem. 2022;13:2880–901.
Bingham NM, Roth PJ. Degradable vinyl copolymers through thiocarbonyl addition-ring-opening (TARO) polymerization. Chem Commun. 2019;55:55–8.
Smith RA, Fu G, McAteer O, Xu M, Gutekunst WR. Radical approach to thioester-containing polymers. J Am Chem Soc. 2019;141:1446–51.
Article CAS PubMed Google Scholar
Kiel GR, Lundberg DJ, Prince E, Husted KEL, Johnson A, Lensch V, et al. Cleavable comonomers for chemically recyclable polystyrene: a general approach to vinyl polymer circularity. J Am Chem Soc. 2022;144:12979–88.
Article CAS PubMed Google Scholar
Ivanchenko O, Mazières S, Harrisson S, Destarac M. Lactide-derived monomers for radical thiocarbonyl addition ring-opening copolymerisation. Polym Chem. 2022;13:5525–9.
Kamiki R, Kubo T, Satoh K. Addition-fragmentation ring-opening polymerization of bio-based thiocarbonyl l-lactide for dual degradable vinyl copolymers. Macromol Rapid Commun. 2023;44:2200537.
Evans RA, Moad G, Rizzardo E, Thang SH. New free-radical ring-opening acrylate monomers. Macromolecules. 1994;27:7935–7.
Article ADS CAS Google Scholar
Evans RA, Rizzardo E. Free-radical ring-opening polymerization of cyclic allylic sulfides. Macromolecules. 1996;29:6983–9.
Article ADS CAS Google Scholar
Paulusse JM, Amir RJ, Evans RA, Hawker CJ. Free-radical polymers with tunable and selective bio- and chemical degradability. J Am Chem Soc. 2009;131:9805–12.
Article CAS PubMed PubMed Central Google Scholar
Huang H, Sun B, Haung Y, Niu J. Radical cascade-triggered controlled ring-opening polymerization of macrocyclic monomers. J Am Chem Soc. 2018;140:10402–6.
Article CAS PubMed Google Scholar
Wang W, Zhou Z, Sathe D, Tang X, Moran S, Jin J, et al. Degradable vinyl random copolymers via photocontrolled radical ring-opening cascade copolymerization. Angew Chem Int Ed. 2022;61:e202113302.
Pięta M, Purohit VB, Paneth P, Pietrasik J, Li L, Plummer CM. An orthogonal O,S-CKA monomer for the introduction of thioester and/or thionoester functionalities by radical polymerization. Polym Chem. 2023;14:3872–80.
Kazama A, Kohsaka Y. Radical polymerization of ‘dehydroaspirin’ with the formation of a hemiacetal ester skeleton: a hint for recyclable vinyl polymers. Polym Chem. 2019;10:2764–8.
Oh XY, Ge Y, Goto A. Synthesis of degradable and chemically recyclable polymers using 4,4-disubstituted five-membered cyclic ketene hemiacetal ester (CKHE) monomers. Chem Sci. 2021;12:13546–56.
Article CAS PubMed PubMed Central Google Scholar
Watanabe H, Kamigaito M. Direct radical copolymerizations of thioamides to generate vinyl polymers with degradable thioether bonds in the backbones. J Am Chem Soc. 2023;145:10948–53.
Article CAS PubMed PubMed Central Google Scholar
Kimura T, Kuroda K, Kubota H, Ouchi M. Metal-catalyzed switching degradation of vinyl polymers via introduction of an “In-Chain” carbon–halogen bonds as the trigger. ACS Macro Lett. 2021;10:1535–9.
Article CAS PubMed Google Scholar
Garrison JB, Hughes RW, Sumerlin BS. Backbone degradation of polymethacrylates via metal-free ambient-temperature photoinduced single-electron transfer. ACS Macro Lett. 2022;11:441–6.
Article CAS PubMed Google Scholar
Makino H, Nishikawa T, Ouchi M. Incorporation of a boryl pendant as the trigger in a methacrylate polymer for backbone degradation. Chem Commun. 2022;5:11957–60.
Yamamoto S, Kubo T, Satoh K. Interlocking degradation of vinyl polymers via main-chain C–C bonds scission by introducing pendant-responsive comonomers. J Polym Sci. 2022;60:3435–46.
Wang HS, Truong NP, Pei Z, Coote ML, Anastasaki A. Reversing RAFT polymerization: near-quantitative monomer generation via a catalyst-free depolymerization approach. J Am Chem Soc. 2022;144:4678–84.
Article CAS PubMed PubMed Central Google Scholar
Jones GR, Wang HS, Parkatzidis K, Whitfield R, Truong NP, Anastasaki A. Reversed controlled polymerization (RCP): depolymerization from well-defined polymers to monomers. J Am Chem Soc. 2023;145:9898–915.
Article CAS PubMed PubMed Central Google Scholar
Curran DP, Shen W. Radical translocation reactions of vinyl radicals: substituent effects on 1,5-hydrogen-transfer reactions. J Am Chem Soc. 1993;115:6051–9.
Nechab M, Mondal S, Bertrand MP. 1,n-Hydrogen-atom transfer (HAT) reactions in which n not equal5: an updated inventory. Chem Eur J. 2014;20:16034–59.
Article CAS PubMed Google Scholar
Sarkar S, Cheung KPS, Gevorgyan V. C-H functionalization reactions enabled by hydrogen atom transfer to carbon-centered radicals. Chem Sci. 2020;11:12974–93.
Article CAS PubMed PubMed Central Google Scholar
Robertson J, Pillai J, Lush RK. Radical translocation reactions in synthesis. Chem Soc Rev. 2001;30:94–103.
Wu X, Ma Z, Feng T, Zhu C. Radical-mediated rearrangements: past, present, and future. Chem Soc Rev. 2021;50:11577–613.
Article CAS PubMed Google Scholar
Moad G, Solomon DH. The chemistry of radical polymerization: second fully revised edition. Oxford: Elsevier; 2006.
Roedel MJ. The molecular structure of polyethylene. I. Chain branching in polyethylene during polymerization. J Am Chem Soc. 1953;75:6110–2.
Axelson DE, Levy GC, Mandelkern L. A quantitative analysis of low-density (branched) polyethylenes by carbon-13 fourier transform nuclear magnetic resonance at 67.9 MHz. Macromolecules. 1979;12:41–52.
Article ADS CAS Google Scholar
Ahmad NM, Heatley F, Lovell PA. Chain transfer to polymer in free-radical solution polymerization of n-Butyl acrylate studied by NMR spectroscopy. Macromolecules. 1998;31:2822–7.
Article ADS CAS Google Scholar
Starnes WH, Schilling FC, Plitz IM, Cais RE, Freed DJ, Hartless RL, et al. Branch structures in poly(vinyl chloride) and the mechanism of chain transfer to monomer during vinyl chloride polymerization. Macromolecules. 1983;16:790–807.
Article ADS CAS Google Scholar
Britton D, Heatley F, Lovell PA. Chain transfer to polymer in free-radical bulk and emulsion polymerization of vinyl acetate studied by NMR spectroscopy. Macromolecules. 1998;31:2828–37.
Article ADS CAS Google Scholar
Sato T, Takahashi H, Tanaka H, Ota T. Radical polymerization of 3-cyano-3-ethoxycarbonylpropyl vinyl ether via addition–abstraction mechanism. J Polym Sci Part A Polym Chem. 1988;26:2839–47.
Article ADS CAS Google Scholar
Sato T, Ito D, Kuki M, Tanaka H, Ota T. Radical polymerization of 3,3-bis(ethoxycarbonyl)propyl vinyl ether via an addition-abstraction mechanism. Macromolecules. 1991;24:2963–7.
Article ADS CAS Google Scholar
Sato T, Nakagawa Y, Kawachi T, Seno M. Radical polymerization of 3,3-dicyanopropyl vinyl ether via an addition-abstraction mechanism. Eur Polym J. 1996;32:827–35.
Sato T, Miki K, Seno M. Radical group-transfer polymerization of 2-thiocyanatoethyl vinyl ether. Macromolecules. 1999;32:4166–72.
Article ADS CAS Google Scholar
Sugihara S, Yoshida A, Kono T, Takayama T, Maeda Y. Controlled radical homopolymerization of representative cationically polymerizable vinyl ethers. J Am Chem Soc. 2019;141:13954–61.
Article CAS PubMed Google Scholar
Sugihara S. From controlled radical polymerization of vinyl ether to polymerization-induced self-assembly. Polym J. 2022;54:1407–18.
Greenley RZ. Free radical copolymerization reactivity ratios. In: Brandrup J, Immergut EH, Grulke EA, Eds. Polymer Handbook. 4th ed. John Wiley & Sons: New York; 1999.
Namikoshi T, Hashimoto T, Urushisaki M. Synthesis of poly(vinyl ether) plastics for optical use by cationic copolymerization of tricyclodecyl Vinyl Ether with n-Butyl Vinyl Ether. J Polym Sci. Part A: Polym Chem. 2007;45:4389–93.
Article ADS CAS Google Scholar
Kozikowski AP, Wu J-P. Protection of alcohols as their (p-methoxybenzyloxy)methyl ethers. Tetrahedron Lett. 1987;28:5125–8.
Andrews RJ, Grulke EA. Glass transition temperatures of polymers. In: Brandrup J, Immergut EH, Grulke EA, Eds. Polymer Handbook. 4th ed. John Wiley & Sons: New York; 1999.
Cullis CF, Hirschler MM. The significance of thermoanalytical measurements in the assessment of polymer flammability. Polymer. 1983;24:834–40.
Satoh K, Nakahara A, Mukunoki K, Sugiyama H, Saito H, Kamigaito M. Sustainable cycloolefin polymer from pine tree oil for optoelectronics material: living cationic polymerization of β-pinene and catalytic hydrogenation of high-molecular-weight hydrogenated poly(β-pinene). Polym Chem. 2014;5:3222–30.
This work was supported by a project (JPNP18016) commissioned by the New Energy and Industrial Technology Development Organization (NEDO). The authors thank Drs. Hironobu Watanabe and Chihiro Homma for fruitful discussions and valuable comments on this research.
Open Access funding provided by Nagoya University.
Department of Molecular and Macromolecular Chemistry, Graduate School of Engineering, Nagoya University, Furo-cho, Chikusa-ku, Nagoya, 464-8603, Japan
Mineto Uchiyama, Masato Imai & Masami Kamigaito
You can also search for this author in PubMed Google Scholar
You can also search for this author in PubMed Google Scholar
You can also search for this author in PubMed Google Scholar
Correspondence to Mineto Uchiyama or Masami Kamigaito.
The authors declare no competing interests.
Publisher’s note Springer Nature remains neutral with regard to jurisdictional claims in published maps and institutional affiliations.
Open Access This article is licensed under a Creative Commons Attribution 4.0 International License, which permits use, sharing, adaptation, distribution and reproduction in any medium or format, as long as you give appropriate credit to the original author(s) and the source, provide a link to the Creative Commons licence, and indicate if changes were made. The images or other third party material in this article are included in the article’s Creative Commons licence, unless indicated otherwise in a credit line to the material. If material is not included in the article’s Creative Commons licence and your intended use is not permitted by statutory regulation or exceeds the permitted use, you will need to obtain permission directly from the copyright holder. To view a copy of this licence, visit http://creativecommons.org/licenses/by/4.0/.
Uchiyama, M., Imai, M. & Kamigaito, M. Synthesis of degradable polymers via 1,5-shift radical isomerization polymerization of vinyl ether derivatives with a cleavable bond. Polym J 56, 359–368 (2024). https://doi.org/10.1038/s41428-023-00869-3
DOI: https://doi.org/10.1038/s41428-023-00869-3
Anyone you share the following link with will be able to read this content:
Sorry, a shareable link is not currently available for this article.
Provided by the Springer Nature SharedIt content-sharing initiative

4-Chlorodehydromethyltestosterone Polymer Journal (Polym J) ISSN 1349-0540 (online) ISSN 0032-3896 (print)