Thank you for visiting nature.com. You are using a browser version with limited support for CSS. To obtain the best experience, we recommend you use a more up to date browser (or turn off compatibility mode in Internet Explorer). In the meantime, to ensure continued support, we are displaying the site without styles and JavaScript.
Nature Chemistry (2024 )Cite this article Electric Relay
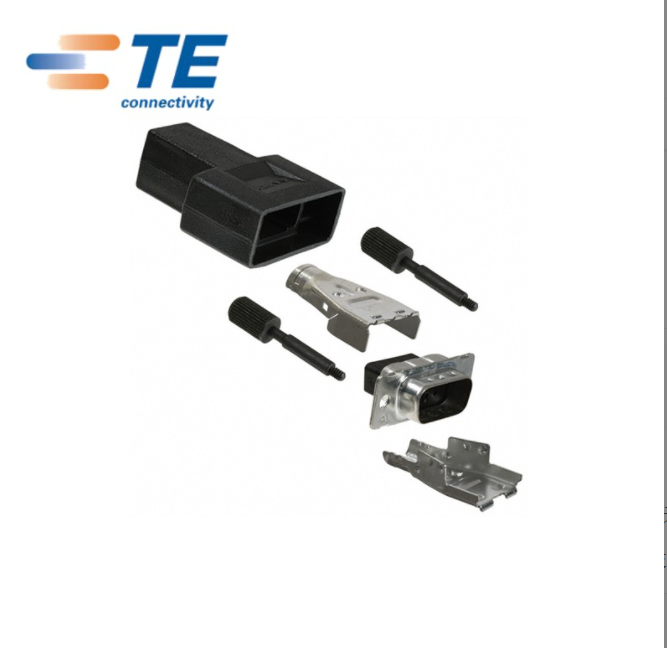
Recent advances in chemical proteomics have focused on developing chemical probes that react with nucleophilic amino acid residues. Although histidine is an attractive candidate due to its importance in enzymatic catalysis, metal binding and protein–protein interaction, its moderate nucleophilicity poses challenges. Its modification is frequently influenced by cysteine and lysine, which results in poor selectivity and narrow proteome coverage. Here we report a singlet oxygen and chemical probe relay labelling method that achieves high selectivity towards histidine. Libraries of small-molecule photosensitizers and chemical probes were screened to optimize histidine labelling, enabling histidine profiling in live cells with around 7,200 unique sites. Using NMR spectroscopy and X-ray crystallography, we characterized the reaction mechanism and the structures of the resulting products. We then applied this method to discover unannotated histidine sites key to enzymatic activity and metal binding in select metalloproteins. This method also revealed the accessibility change of histidine mediated by protein–protein interaction that influences select protein subcellular localization, underscoring its capability in discovering functional histidines.
This is a preview of subscription content, access via your institution
Access Nature and 54 other Nature Portfolio journals
Get Nature+, our best-value online-access subscription
Receive 12 print issues and online access
Prices may be subject to local taxes which are calculated during checkout
The mass spectrometry data generated in this study have been deposited to the ProteomeXchange Consortium via the iProX (ref. 82) partner repository with the dataset identifier PXD042377 (Histidine_Profiling_MS dataset). Crystallographic data for small molecule 7 reported in this Article have been deposited at the Cambridge Crystallographic Data Centre, under deposition number CCDC 2312673. Copies of the data can be obtained free of charge via https://www.ccdc.cam.ac.uk/structures. The dataset corresponding to the Python and R codes are available via Zenodo at https://doi.org/10.5281/zenodo.10867769 (ref. 83) or from the corresponding author upon request. Source data are provided with this paper.
The Python code used for cleaning up histidine-containing peptides data, SASA analysis, secondary structure distribution analysis and distance measurement, along with the R code for domain enrichment analysis, are available via Zenodo at https://doi.org/10.5281/zenodo.10867769 (ref. 83) or from the corresponding author upon request.
Liu, Y., Patricelli, M. P. & Cravatt, B. F. Activity-based protein profiling: the serine hydrolases. Proc. Natl Acad. Sci. USA 96, 14694–14699 (1999).
Article CAS PubMed PubMed Central Google Scholar
Cravatt, B. F., Wright, A. T. & Kozarich, J. W. Activity-based protein profiling: from enzyme chemistry to proteomic chemistry. Annu. Rev. Biochem. 77, 383–414 (2008).
Article CAS PubMed Google Scholar
Long, J. Z. & Cravatt, B. F. The metabolic serine hydrolases and their functions in mammalian physiology and disease. Chem. Rev. 111, 6022–6063 (2011).
Article CAS PubMed PubMed Central Google Scholar
Kidd, D., Liu, Y. & Cravatt, B. F. Profiling serine hydrolase activities in complex proteomes. Biochemistry 40, 4005–4015 (2001).
Article CAS PubMed Google Scholar
Patricelli, M. P. et al. Functional interrogation of the kinome using nucleotide acyl phosphates. Biochemistry 46, 350–358 (2007).
Article CAS PubMed Google Scholar
Zhao, Q. et al. Broad-spectrum kinase profiling in live cells with lysine-targeted sulfonyl fluoride probes. J. Am. Chem. Soc. 139, 680–685 (2017).
Article CAS PubMed PubMed Central Google Scholar
Kato, D. et al. Activity-based probes that target diverse cysteine protease families. Nat. Chem. Biol. 1, 33–38 (2005).
Article CAS PubMed Google Scholar
Saghatelian, A., Jessani, N., Joseph, A., Humphrey, M. & Cravatt, B. F. Activity-based probes for the proteomic profiling of metalloproteases. Proc. Natl Acad. Sci. USA 101, 10000–10005 (2004).
Article CAS PubMed PubMed Central Google Scholar
Vocadlo, D. J. & Bertozzi, C. R. A strategy for functional proteomic analysis of glycosidase activity from cell lysates. Angew. Chem. Int. Ed. 43, 5338–5342 (2004).
Hekmat, O., Kim, Y. W., Williams, S. J., He, S. & Withers, S. G. Active-site peptide “fingerprinting” of glycosidases in complex mixtures by mass spectrometry. Discovery of a novel retaining beta-1,4-glycanase in Cellulomonas fimi. J. Biol. Chem. 280, 35126–35135 (2005).
Article CAS PubMed Google Scholar
Kumar, S. et al. Activity-based probes for protein tyrosine phosphatases. Proc. Natl Acad. Sci. USA 101, 7943–7948 (2004).
Article CAS PubMed PubMed Central Google Scholar
Bachovchin, D. A., Brown, S. J., Rosen, H. & Cravatt, B. F. Identification of selective inhibitors of uncharacterized enzymes by high-throughput screening with fluorescent activity-based probes. Nat. Biotechnol. 27, 387–394 (2009).
Article CAS PubMed PubMed Central Google Scholar
Nomura, D. K. et al. Monoacylglycerol lipase regulates a fatty acid network that promotes cancer pathogenesis. Cell 140, 49–61 (2010).
Article CAS PubMed PubMed Central Google Scholar
Weerapana, E. et al. Quantitative reactivity profiling predicts functional cysteines in proteomes. Nature 468, 790–795 (2010).
Article CAS PubMed PubMed Central Google Scholar
Hacker, S. M. et al. Global profiling of lysine reactivity and ligandability in the human proteome. Nat. Chem. 9, 1181–1190 (2017).
Article CAS PubMed PubMed Central Google Scholar
Hahm, H. S. et al. Global targeting of functional tyrosines using sulfur-triazole exchange chemistry. Nat. Chem. Biol. 16, 150–160 (2020).
Article CAS PubMed Google Scholar
Lin, S. X. et al. Redox-based reagents for chemoselective methionine bioconjugation. Science 355, 597–602 (2017).
Article CAS PubMed PubMed Central Google Scholar
Ma, N. et al. 2H-Azirine-based reagents for chemoselective bioconjugation at carboxyl residues inside live cells. J. Am. Chem. Soc. 142, 6051–6059 (2020).
Article CAS PubMed Google Scholar
Bach, K., Beerkens, B. L. H., Zanon, P. R. A. & Hacker, S. M. Light-activatable, 2,5-disubstituted tetrazoles for the proteome-wide profiling of aspartates and glutamates in living bacteria. ACS Cent. Sci. 6, 546–554 (2020).
Article CAS PubMed PubMed Central Google Scholar
Gutteridge, A. & Thornton, J. M. Understanding nature’s catalytic toolkit. Trends Biochem. Sci. 30, 622–629 (2005).
Article CAS PubMed Google Scholar
Dokmanic, I., Sikic, M. & Tomic, S. Metals in proteins: correlation between the metal-ion type, coordination number and the amino-acid residues involved in the coordination. Acta Crystallogr. D Biol. Crystallogr. 64, 257–263 (2008).
Article CAS PubMed Google Scholar
Martinez-Fabregas, J., Rubio, S., Diaz-Quintana, A., Diaz-Moreno, I. & De la Rosa, M. A. Proteomic tools for the analysis of transient interactions between metalloproteins. FEBS J. 278, 1401–1410 (2011).
Article CAS PubMed Google Scholar
Parsons, W. H. et al. AIG1 and ADTRP are atypical integral membrane hydrolases that degrade bioactive FAHFAs. Nat. Chem. Biol. 12, 367–372 (2016).
Article CAS PubMed PubMed Central Google Scholar
Watanabe, H. et al. Histidine-mediated intramolecular electrostatic repulsion for controlling pH-dependent protein–protein interaction. ACS Chem. Biol. 14, 2729–2736 (2019).
Article CAS PubMed Google Scholar
Hindupur, S. K. et al. The protein histidine phosphatase LHPP is a tumour suppressor. Nature 555, 678–682 (2018).
Article CAS PubMed PubMed Central Google Scholar
Srivastava, S. et al. Histidine phosphorylation relieves copper inhibition in the mammalian potassium channel KCa3.1. Elife 5, e16093 (2016).
Article PubMed PubMed Central Google Scholar
Wilke, K. E., Francis, S. & Carlson, E. E. Activity-based probe for histidine kinase signaling. J. Am. Chem. Soc. 134, 9150–9153 (2012).
Article CAS PubMed PubMed Central Google Scholar
Jia, S., He, D. & Chang, C. J. Bioinspired thiophosphorodichloridate reagents for chemoselective histidine bioconjugation. J. Am. Chem. Soc. 141, 7294–7301 (2019).
Article CAS PubMed PubMed Central Google Scholar
Takaoka, Y., Tsutsumi, H., Kasagi, N., Nakata, E. & Hamachi, I. One-pot and sequential organic chemistry on an enzyme surface to tether a fluorescent probe at the proximity of the active site with restoring enzyme activity. J. Am. Chem. Soc. 128, 3273–3280 (2006).
Article CAS PubMed Google Scholar
Li, J. et al. ACR-based probe for the quantitative profiling of histidine reactivity in the human proteome. J. Am. Chem. Soc. 145, 5252–5260 (2023).
Article CAS PubMed Google Scholar
Wan, C. et al. Histidine-specific bioconjugation via visible-light-promoted thioacetal activation. Chem. Sci. 13, 8289–8296 (2022).
Article CAS PubMed PubMed Central Google Scholar
Chen, X. et al. Histidine-specific peptide modification via visible-light-promoted C–H alkylation. J. Am. Chem. Soc. 141, 18230–18237 (2019).
Article CAS PubMed Google Scholar
Noisier, A. F. M. et al. Late-stage functionalization of histidine in unprotected peptides. Angew. Chem. Int. Ed. 58, 19096–19102 (2019).
Nakane, K. et al. Proximity histidine labeling by umpolung strategy using singlet oxygen. J. Am. Chem. Soc. 143, 7726–7731 (2021).
Article CAS PubMed Google Scholar
Zhai, Y. et al. Spatiotemporal-resolved protein networks profiling with photoactivation dependent proximity labeling. Nat. Commun. 13, 4906 (2022).
Article CAS PubMed PubMed Central Google Scholar
Zhao, X., Liu, J., Fan, J., Chao, H. & Peng, X. Recent progress in photosensitizers for overcoming the challenges of photodynamic therapy: from molecular design to application. Chem. Soc. Rev. 50, 4185–4219 (2021).
Article CAS PubMed Google Scholar
Xu, W. et al. Three-pronged attack by homologous far-red/NIR AIEgens to achieve 1+1+1>3 synergistic enhanced photodynamic therapy. Angew. Chem. Int. Ed. 59, 9610–9616 (2020).
Luo, H. et al. Photocatalytic chemical crosslinking for profiling RNA-protein interactions in living cells. Angew. Chem. Int. Ed. 61, e202202008 (2022).
Kitamura, T., Nakata, H., Takahashi, D. & Toshima, K. Hypocrellin B-based activatable photosensitizers for specific photodynamic effects against high H2O2-expressing cancer cells. Chem. Commun. 58, 242–245 (2021).
Baier, J. et al. Singlet oxygen generation by UVA light exposure of endogenous photosensitizers. Biophys. J. 91, 1452–1459 (2006).
Article CAS PubMed PubMed Central Google Scholar
Fang, Y. & Zou, P. Photocatalytic proximity labeling for profiling the subcellular organization of biomolecules. ChemBioChem 24, e202200745 (2023).
Article CAS PubMed Google Scholar
Tamura, T., Takato, M., Shiono, K. & Hamachi, I. Development of a photoactivatable proximity labeling method for the identification of nuclear proteins. Chem. Lett. 49, 145–148 (2020).
Liu, H. et al. Antigen-specific T cell detection via photocatalytic proximity cell labeling (PhoXCELL). J. Am. Chem. Soc. 144, 5517–5526 (2022).
Article CAS PubMed Google Scholar
Xu, F. et al. Hypoxia-activated NIR photosensitizer anchoring in the mitochondria for photodynamic therapy. Chem. Sci. 10, 10586–10594 (2019).
Article CAS PubMed PubMed Central Google Scholar
Ma, H. et al. New Cy5 photosensitizers for cancer phototherapy: a low singlet–triplet gap provides high quantum yield of singlet oxygen. Chem. Sci. 12, 13809–13816 (2021).
Article CAS PubMed PubMed Central Google Scholar
Bauer, D., Montforts, F. P., Losi, A. & Gorner, H. Photoprocesses of chlorin e6 glucose derivatives. Photochem. Photobiol. Sci. 11, 925–930 (2012).
Article CAS PubMed Google Scholar
Liu, S., Feng, G., Tang, B. Z. & Liu, B. Recent advances of AIE light-up probes for photodynamic therapy. Chem. Sci. 12, 6488–6506 (2021).
Article CAS PubMed PubMed Central Google Scholar
Hu, F., Xu, S. & Liu, B. Photosensitizers with aggregation-induced emission: materials and biomedical applications. Adv. Mater. 30, e1801350 (2018).
Wang, P. et al. Mapping spatial transcriptome with light-activated proximity-dependent RNA labeling. Nat. Chem. Biol. 15, 1110–1119 (2019).
Article CAS PubMed Google Scholar
Toh, K. et al. Chemoproteomic identification of blue-light-damaged proteins. J. Am. Chem. Soc. 144, 20171–20176 (2022).
Article CAS PubMed Google Scholar
Hananya, N., Ye, X., Koren, S. & Muir, T. W. A genetically encoded photoproximity labeling approach for mapping protein territories. Proc. Natl Acad. Sci. USA 120, e2219339120 (2023).
Article CAS PubMed PubMed Central Google Scholar
Weerapana, E., Speers, A. E. & Cravatt, B. F. Tandem orthogonal proteolysis-activity-based protein profiling (TOP-ABPP)—a general method for mapping sites of probe modification in proteomes. Nat. Protoc. 2, 1414–1425 (2007).
Article CAS PubMed Google Scholar
Kong, A. T., Leprevost, F. V., Avtonomov, D. M., Mellacheruvu, D. & Nesvizhskii, A. I. MSFragger: ultrafast and comprehensive peptide identification in mass spectrometry-based proteomics. Nat. Methods 14, 513–520 (2017).
Article CAS PubMed PubMed Central Google Scholar
Pattison, D. I., Rahmanto, A. S. & Davies, M. J. Photo-oxidation of proteins. Photochem. Photobiol. Sci. 11, 38–53 (2012).
Article CAS PubMed Google Scholar
Grassi, L. & Cabrele, C. Susceptibility of protein therapeutics to spontaneous chemical modifications by oxidation, cyclization, and elimination reactions. Amino Acids 51, 1409–1431 (2019).
Article CAS PubMed Google Scholar
Oslund, R. C. et al. Detection of cell–cell interactions via photocatalytic cell tagging. Nat. Chem. Biol. 18, 850–858 (2022).
Article CAS PubMed Google Scholar
Ryu, K. A., Kaszuba, C. M., Bissonnette, N. B., Oslund, R. C. & Fadeyi, O. O. Interrogating biological systems using visible-light-powered catalysis. Nat. Rev. Chem. 5, 322–337 (2021).
Article CAS PubMed Google Scholar
Cao, J. et al. Multiplexed CuAAC Suzuki–Miyaura labeling for tandem activity-based chemoproteomic profiling. Anal. Chem. 93, 2610–2618 (2021).
Article CAS PubMed PubMed Central Google Scholar
Marconi, G. & Quintana, R. Methylene blue dyeing of cellular nuclei during salpingoscopy, a new in-vivo method to evaluate vitality of tubal epithelium. Hum. Reprod. 13, 3414–3417 (1998).
Article CAS PubMed Google Scholar
Muller, M. et al. Light-mediated discovery of surfaceome nanoscale organization and intercellular receptor interaction networks. Nat. Commun. 12, 7036 (2021).
Article CAS PubMed PubMed Central Google Scholar
Singha Roy, S. J. et al. Photoredox-catalyzed labeling of hydroxyindoles with chemoselectivity (PhotoCLIC) for site-specific protein bioconjugation. Angew. Chem. Int. Ed. 62, e202300961 (2023).
Zheng, F., Yu, C., Zhou, X. & Zou, P. Genetically encoded photocatalytic protein labeling enables spatially-resolved profiling of intracellular proteome. Nat. Commun. 14, 2978 (2023).
Article CAS PubMed PubMed Central Google Scholar
Agon, V. V., Bubb, W. A., Wright, A., Hawkins, C. L. & Davies, M. J. Sensitizer-mediated photooxidation of histidine residues: evidence for the formation of reactive side-chain peroxides. Free Radic. Biol. Med. 40, 698–710 (2006).
Article CAS PubMed Google Scholar
Varadi, M. et al. AlphaFold Protein Structure Database: massively expanding the structural coverage of protein-sequence space with high-accuracy models. Nucleic Acids Res. 50, D439–D444 (2022).
Article CAS PubMed Google Scholar
Jumper, J. et al. Highly accurate protein structure prediction with AlphaFold. Nature 596, 583–589 (2021).
Article CAS PubMed PubMed Central Google Scholar
Backus, K. M. et al. Proteome-wide covalent ligand discovery in native biological systems. Nature 534, 570–574 (2016).
Article CAS PubMed PubMed Central Google Scholar
Abbasov, M. E. et al. A proteome-wide atlas of lysine-reactive chemistry. Nat. Chem. 13, 1081–1092 (2021).
Article CAS PubMed PubMed Central Google Scholar
Cheng, Y. et al. Co-evolution-based prediction of metal-binding sites in proteomes by machine learning. Nat. Chem. Biol. 19, 548–555 (2023).
Article CAS PubMed Google Scholar
Klein, D. J., Moore, P. B. & Steitz, T. A. The contribution of metal ions to the structural stability of the large ribosomal subunit. RNA 10, 1366–1379 (2004).
Article CAS PubMed PubMed Central Google Scholar
Chan, Y. L., Suzuki, K., Olvera, J. & Wool, I. G. Zinc finger-like motifs in rat ribosomal proteins S27 and S29. Nucleic Acids Res. 21, 649–655 (1993).
Article CAS PubMed PubMed Central Google Scholar
Rivlin, A. A., Chan, Y. L. & Wool, I. G. The contribution of a zinc finger motif to the function of yeast ribosomal protein YL37a. J. Mol. Biol. 294, 909–919 (1999).
Article CAS PubMed Google Scholar
Dang, L. et al. Cancer-associated IDH1 mutations produce 2-hydroxyglutarate. Nature 462, 739–744 (2009).
Article CAS PubMed PubMed Central Google Scholar
Perez-Alvarado, G. C. et al. Structure of the cysteine-rich intestinal protein, CRIP. J. Mol. Biol. 257, 153–174 (1996).
Article CAS PubMed Google Scholar
Imberechts, D. & Vandenberghe, W. Defects in PINK-PRKN-PARK7/DJ-1-dependent mitophagy and autosomal recessive Parkinson disease. Autophagy 19, 1872–1873 (2022).
Dolgacheva, L. P., Berezhnov, A. V., Fedotova, E. I., Zinchenko, V. P. & Abramov, A. Y. Role of DJ-1 in the mechanism of pathogenesis of Parkinson’s disease. J. Bioenerg. Biomembr. 51, 175–188 (2019).
Article CAS PubMed PubMed Central Google Scholar
Hu, S. et al. Molecular chaperones and Parkinson’s disease. Neurobiol. Dis. 160, 105527 (2021).
Article CAS PubMed Google Scholar
Zhang, X. et al. An effective synthetic entry to fused benzimidazoles via iodocyclization. Adv. Synth. Catal. 353, 1429–1437 (2011).
Kabsch, W. & Sander, C. Dictionary of protein secondary structure: pattern recognition of hydrogen-bonded and geometrical features. Biopolymers 22, 2577–2637 (1983).
Article CAS PubMed Google Scholar
Joosten, R. P. et al. A series of PDB related databases for everyday needs. Nucleic Acids Res. 39, D411–D419 (2011).
Article CAS PubMed Google Scholar
Savojardo, C., Manfredi, M., Martelli, P. L. & Casadio, R. Solvent accessibility of residues undergoing pathogenic variations in humans: from protein structures to protein sequences. Front. Mol. Biosci. 7, 626363 (2020).
Article CAS PubMed Google Scholar
Mi, H., Muruganujan, A., Casagrande, J. T. & Thomas, P. D. Large-scale gene function analysis with the PANTHER classification system. Nat. Protoc. 8, 1551–1566 (2013).
Article PubMed PubMed Central Google Scholar
Ma, J. et al. iProX: an integrated proteome resource. Nucleic Acids Res. 47, D1211–D1217 (2019).
Li, G. Global profiling of functional histidines in live cells using small molecule photosensitizer and chemical probe relay labeling. Zenodo https://doi.org/10.5281/zenodo.10867769 (2024).
We thank the mass spectrometry, imaging, sequencing and NMR core facility in Shenzhen Bay Laboratory for their assistance in running samples and collecting data. We thank X. Li, Y. Liu, C. Wang and W. Zhong for helpful discussion and proofreading assistance. We thank Z. Li (Peking University Shenzhen Graduate School) for providing the thioacetal alkyne (TAA) probe. We are grateful for financial support of this work from Shenzhen Bay Laboratory Startup (21240041 to G.L.) and Grant from Shenzhen Bay Laboratory Open Fund (SZBL2020090501008 to G.L.). The funders had no role in study design, data collection and analysis, decision to publish or preparation of the manuscript.
Institute of Systems and Physical Biology, Shenzhen Bay Laboratory, Shenzhen, China
Yansheng Zhai, Xinyu Zhang, Lin Zhu, Zhe Zhang, Kailu Tian, Yan Huang, Xi Yang & Gang Li
State Key Laboratory of Crop Stress Biology for Arid Areas, College of Life Sciences, Northwest A & F University, Yangling, China
Key Laboratory of Bioorganic Chemistry and Molecular Engineering, Ministry of Education and Beijing National Laboratory for Molecular Science, College of Chemistry and Molecular Engineering, Peking University, Beijing, China
Zijing Chen & Tuoping Luo
Dingyuan Yan & Dong Wang
School of Life Sciences, University of Science and Technology of China, Hefei, China
Synthetic and Functional Biomolecules Center, Beijing National Laboratory for Molecular Sciences, Key Laboratory of Bioorganic Chemistry and Molecular Engineering of Ministry of Education, College of Chemistry and Molecular Engineering, Peking University, Beijing, China
State Key Laboratory of Fine Chemicals, Frontiers Science Center for Smart Materials Oriented Chemical Engineering, Dalian University of Technology, Dalian, China
Institute of Molecular Physiology, Shenzhen Bay Laboratory, Shenzhen, China
Yu-Hsuan Tsai & Tuoping Luo
Peking-Tsinghua Center for Life Sciences, Academy for Advanced Interdisciplinary Studies, Peking University, Beijing, China
You can also search for this author in PubMed Google Scholar
You can also search for this author in PubMed Google Scholar
You can also search for this author in PubMed Google Scholar
You can also search for this author in PubMed Google Scholar
You can also search for this author in PubMed Google Scholar
You can also search for this author in PubMed Google Scholar
You can also search for this author in PubMed Google Scholar
You can also search for this author in PubMed Google Scholar
You can also search for this author in PubMed Google Scholar
You can also search for this author in PubMed Google Scholar
You can also search for this author in PubMed Google Scholar
You can also search for this author in PubMed Google Scholar
You can also search for this author in PubMed Google Scholar
You can also search for this author in PubMed Google Scholar
You can also search for this author in PubMed Google Scholar
All authors reviewed the manuscript. G.L. conceived and supervised the research. Y.Z., X.Z., X.W., Y.H., Y.-H.T. and G.L. designed and analysed biological experiments. Z.C., L.Z., T.L. and G.L. designed and analysed chemical experiments. D.Y., W.S. and D.W. provided the photosensitizers. X.Y. wrote the Python program for data processing and generated the figures. K.T. conducted the domain enrichment analysis. Z.Z. performed the solvent-accessible surface area analysis, secondary structure distribution analysis and distance measurement between histidines and active sites. Y.Z. and G.L. wrote the manuscript with input from all the authors.
The authors declare no competing interests.
Nature Chemistry thanks Shinichi Sato and the other, anonymous, reviewer(s) for their contribution to the peer review of this work.
Publisher’s note Springer Nature remains neutral with regard to jurisdictional claims in published maps and institutional affiliations.
a) Structures of small molecule photosensitizers. Eosin B (EB); Eosin Y (EY); Hypocrellin A (HA); Hypocrellin B (HB); Riboflavin (RF); Rose Bengal (RB); Dibromofluorescein (DBF); TCy5-CHO (T5C); TCy5-Btz (T5B); TCy5-Ph-3F (T5P); Methylene blue (MB); Icy-OH (IO); Methyl pyropheophorbide-a (MP); Chlorin e6 trimethyl ester (CE); TTPy-alkyne (TA); TTPy-OH (TO); DPA-SCPI (DS); b) Structures of chemical probes. N-(2-aminophenyl)pent-4-ynamide (NPA); Propargylamine (PA); 2-ethynylaniline (2-EA); 3-ethynylaniline (3-EA); 4-ethynylaniline (4-EA); 2-ethynylphenol (2-EP); 3-ethynylphenol (3-EP); 4-ethynylphenol (4-EP); 3-ethynyl-4-methylaniline (3E4MA); 3-ethynyl-4-fluoroaniline (3E-4FA); 5-ethynyl-2-fluoroaniline (5E-2FA); thioacetal alkyne (TAA); 3-ethynyl-N-methylaniline (3E-MA); 3-ethynylpyrazin-2-amine (3EP-2A); 5-ethynylpyridin-3-amine (5EP-3A); 4-ethynylpiperidine (4-EPD).
a) The distribution analysis of the PSMs for different photosensitizers in the open search. b) The distribution of singlet oxygen-sensitive amino acids in enriched peptide and human proteome. c) Labeling sites in closed search, where histidine (H) and one of the other 19 amino acids, as well as the N or C protein terminal, were jointly searched with differential masses of 229 and 247 Da. d) Box plot of percentage of histidine sites for combinations in c. The center line shows the median, while the box hinges mark the first and third quartiles. Whiskers indicate the full data range. n = 21 closed search analyses.
X-ray structure of the acyl-histamine oxidation product 7 (CCDC 2312673).
a) Histogram plot showing the distribution of distances of non-identified histidine sites to the active sites for the identified proteins. b) Calculation of the proportions of identified histidine sites, non-identified histidine sites, and histidine residues in the active site for the identified proteins, as well as the proportion of histidine residues in the active site for the human proteome. c) The distribution of solvent accessibility of non-identified histidine residues in the identified proteins. d) The distribution of solvent accessibility of histidine residues in human proteome. e) The secondary structures analysis of the distribution of non-identified histidine sites in the identified proteome. f) The secondary structures analysis of the distribution of histidine residues in human proteome.
a) Forward and reverse SILAC experiments were used to identify the metal-binding dependent histidine sites using the three additional photosensitizers: T5C, T5B, TO. b) Venn diagram illustrating the overlapping proteins identified with different photosensitizers.
a) Proteins consistently exhibiting over 1.5-fold increase in at least two forward and reverse experiments were listed. The overlapping proteins between forward and reverse experiments were selected, indicating their increased interaction with PARK7 after mitophagy. b) Western blot analysis confirming the knockdown of LUC7L3, BAG2, TMOD3, PLEC. c) Real-time PCR analysis was performed for BANF1 knockdown due to the unavailability of a suitable antibody. Data are presented as mean values +/− SD. n = 3 biologically independent experiments.
Statistical source data of supplementary figures.
Statistical source data of Fig. 2.
Statistical source data of Fig. 4.
Unprocessed western blots and gels; statistical source data of Fig. 5.
Unprocessed western blots and gels; statistical source data of Fig. 6.
Statistical source data of Extended Data Fig. 2.
Statistical source data of Extended Data Fig. 4.
Statistical source data of Extended Data Fig. 5.
Unprocessed western blots and statistical source data of Extended Data Fig. 6.
Springer Nature or its licensor (e.g. a society or other partner) holds exclusive rights to this article under a publishing agreement with the author(s) or other rightsholder(s); author self-archiving of the accepted manuscript version of this article is solely governed by the terms of such publishing agreement and applicable law.
Zhai, Y., Zhang, X., Chen, Z. et al. Global profiling of functional histidines in live cells using small-molecule photosensitizer and chemical probe relay labelling. Nat. Chem. (2024). https://doi.org/10.1038/s41557-024-01545-6
DOI: https://doi.org/10.1038/s41557-024-01545-6
Anyone you share the following link with will be able to read this content:
Sorry, a shareable link is not currently available for this article.
Provided by the Springer Nature SharedIt content-sharing initiative
Nature Chemistry (Nat. Chem.) ISSN 1755-4349 (online) ISSN 1755-4330 (print)
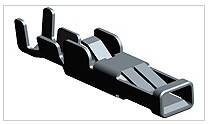
Harness Sign up for the Nature Briefing: Translational Research newsletter — top stories in biotechnology, drug discovery and pharma.