Researchers are developing an "intelligent carpet" for VR locomotion, that could be useful in gaming, healthcare and fitness.
Researchers from the Gwangju Institute of Science and Technology (GIST) in Korea and MIT CSAIL have developed "Seamless-walk," a groundbreaking foot-based virtual reality locomotion system. It promises a natural and comfortable experience without the need for cumbersome walking equipment (e.g., Kat VR) or body pose video recordings. Adult Gait Trainer
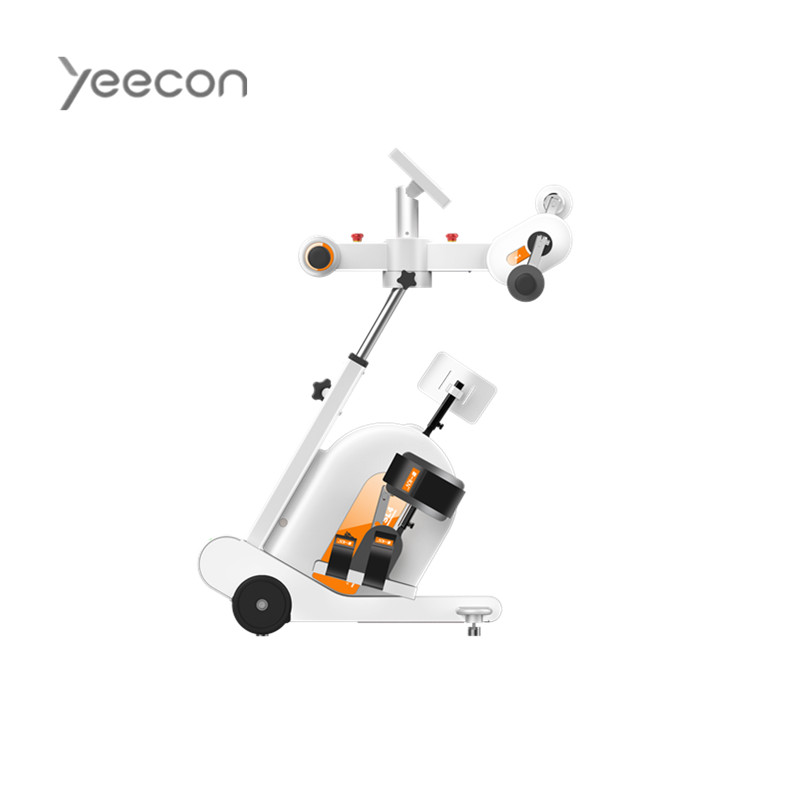
Dr. Kyung-Joong Kim, Associate Professor at GIST, explained, "When we started collaborating with MIT, they introduced an interesting new sensor called the 'intelligent carpet.' We decided to develop a VR game controller with the 'intelligent carpet' sensor that would be useful in VR gaming."
"Seamless-walk" employs the "intelligent carpet" sensor to capture high-resolution foot pressure imprints in real-time. The pressure data is then processed by a machine learning model using K-means clustering, which separates the pressure points into two clusters corresponding to the user's left and right feet. The user's body direction and foot intervals are subsequently determined to estimate movement speed and angle.
Check your inbox or spam folder to confirm your subscription.
The modular structure of "Seamless-walk" allows for scalable and cost-effective installation of a touch sensing platform. During tests involving 80 participants, the system demonstrated an immersive, natural, and comfortable VR experience, outperforming existing VR locomotion methods.
Dr. Kim envisions future applications for "Seamless-walk" in healthcare and fitness industries. "In the long run, we believe that our technology could be used in healthcare. 'Seamless-walk' is not only a VR gamepad but also a gait recognition and analysis method," said Dr. Kim. The team plans to add more detailed gait analysis functions to the system, which could be used for fall detection, health monitoring, and gym-based assessments.
Note: Links to online stores in articles can be so-called affiliate links. If you buy through this link, MIXED receives a commission from the provider. For you the price does not change.

Physical Therapy Check your inbox or spam folder to confirm your subscription.