Thank you for visiting nature.com. You are using a browser version with limited support for CSS. To obtain the best experience, we recommend you use a more up to date browser (or turn off compatibility mode in Internet Explorer). In the meantime, to ensure continued support, we are displaying the site without styles and JavaScript.
Nature Communications volume 15, Article number: 3510 (2024 ) Cite this article Low Voltage Linear Actuator
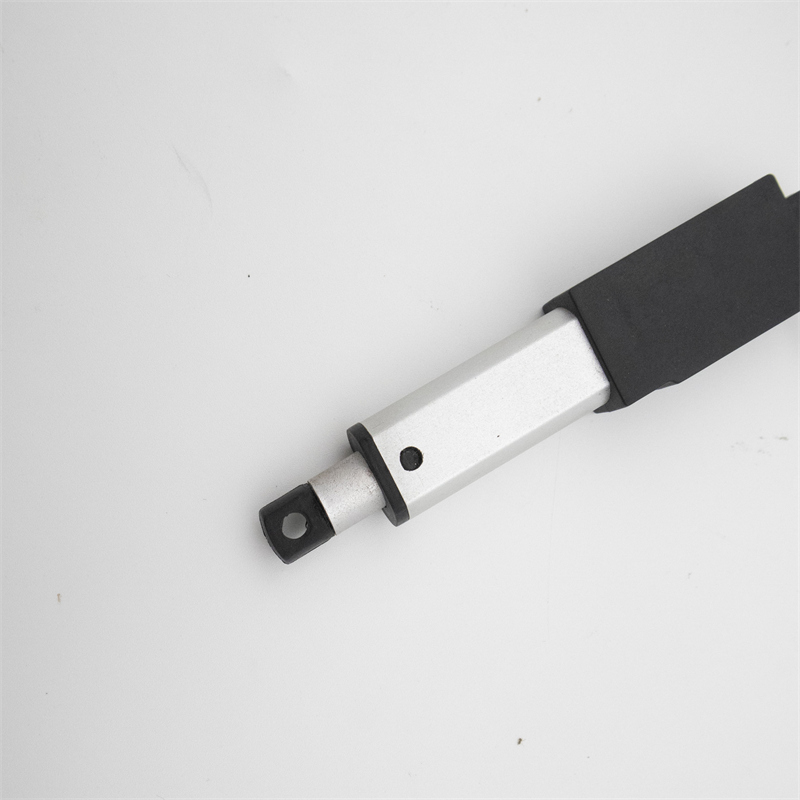
Soft actuators produce the mechanical force needed for the functional movements of soft robots, but they suffer from critical drawbacks since previously reported soft actuators often rely on electrical wires or pneumatic tubes for the power supply, which would limit the potential usage of soft robots in various practical applications. In this article, we review the new types of untethered soft actuators that represent breakthroughs and discuss the future perspective of soft actuators. We discuss the functional materials and innovative strategies that gave rise to untethered soft actuators and deliver our perspective on challenges and opportunities for future-generation soft actuators.
The recent advances in materials and innovative design architectures served to accelerate the development of soft actuators that provide the source of power for the locomotion of the robots. Unlike traditional robots that rely on rigid and bulky structures, soft robots are composed of materials that mimic biological tissues, such as elastomers or hydrogels, enabling them to mechanically deform like living organisms. Their flexible nature grants soft robots the ability to navigate complex environments, manipulate delicate objects, and interact safely with humans. Despite the revolutionary properties of soft robots and their potential to transform a variety of robotics industries, there exist critical technological challenges that soft robotics need to address. The technological limitations arise from the resultant weak actuation force to produce effective locomotion of robots, resulting in slow mobility and trivial force translation. However, a variety of technical and design breakthroughs have offered viable solutions to supply the power source and reinforce actuation force. Many of the recent soft robots resort to electrical wires and fluid tubes to supply an adequate power source for actuation1,2. Also, the recent strides in materials and mechanical design played a pivotal role in amplifying actuation force, exemplified by the incorporation of materials with variable stiffness and phase change materials3,4.
Yet, the most problematic hurdle, which even affects the overall performance and potential applications of soft robots, arises from the external wiring. Here, wires or wiring mean the external tether that connects the soft robot and the power source for the power supply: they do not refer to the electric wires inside the soft robot. A great number of the existing soft robots often rely on bulky pneumatic pumps or complex electrical wiring to supply the driving force for soft actuators via external power sources. Prior works on soft robots usually utilize the pump to create pressure or fluid flow, which drives the mechanical motion of the actuator, and electric motors can also generate mechanical motion with the electrical input. However, the incorporation of wires for the power source circumscribes the functionality and movement of soft robots despite the favorable characteristics of soft robots. For instance, when navigating through a tortuous path or reaching a site to which human beings do not access, the pneumatic tubes or wires exert a tension force on the robots and hinder their physical movements. In this regard, recent studies reported new types of soft actuators that do not accompany pneumatic pumps or electrical wirings to enhance the mobility of the soft robots and also to endow the robots with versatile functionalities.
For pneumatic actuators, the pneumatic pumps serve an essential role in generating a mechanical force by using compressed gas or moving the liquid for the rapid fluid pressure increase. Yet, the incorporation of the pneumatic pump into the soft robotics would impair the mobility and the core functionalities of the soft robots because the pumps are usually relatively bulky and heavy when compared to the soft robots themselves. To address this issue, several recent studies demonstrated pump-less pneumatic actuation by employing the phase change materials that generate the volume change as the materials switch between liquid and gaseous states, thus resulting in the inflation and deflation of actuators. Here, the pump-less pneumatic actuators can be defined as the soft actuators that do not use the actual pump but generate a pneumatic force by the phase change of material just as if utilizing the pneumatic pump. In other words, the pump-less pneumatic actuators just reproduce the end effect of the pump by a different working mechanism without using the actual pump. The absence of the pneumatic pump in the robotic design also eliminates the need for pneumatic tubes to infuse/extract air into/from the actuator, thereby making the design completely untethered.
Likewise, external stimuli can deliver a considerable amount of mechanical displacement and force needed to actuate the soft robots in an untethered manner: the external stimuli in this article include magnetic field, heat, electricity, light, and humidity. Hence, without physically connecting the electrical tethering to the soft actuators to provide the power source, the external stimuli can enable the soft actuator to produce mechanical displacement since the materials are designed to actuate as programmed. As opposed to the pneumatics-based soft actuators that require the onboard power source (such as a battery or self-powering energy harvesting devices) to supply power to induce pneumatic force, some of these actuators receive the power to induce mechanical displacement in a completely untethered fashion. For example, systematic manipulation of a magnetic field can control the movement of the magnet-driven soft actuator as intended without any type of wiring. Similarly, if the antennas are incorporated into the soft robotic system, electromagnetic waves can be utilized to provide power wirelessly to operate the soft actuators5,6,7,8, or it also enables the remote control of the actuators via wireless communication9,10 Therefore, external stimuli-driven soft actuators retain the potential to represent the breakthrough in the field of soft robotics although there exist considerable limitations to be resolved. In this light, it would be a highly valuable resource to introduce untethered soft actuators and discuss the future perspective of new types of soft robots. There are a considerable number of review articles on soft actuators and robotics11,12,13,14,15. However, no review paper has dealt with recent advances in untethered soft actuators for soft robotics that demonstrated meaningful outcomes within a few years. Recently, roboticists and researchers proposed an explosive number of soft actuators for soft robots based on innovative structural designs and functional materials that represent breakthroughs in the field of soft robotics. Furthermore, as the field of soft actuators is relatively new and drawing a substantial amount of interest in the related fields, there exists a demand for an article that systematically reviews the current trend and informs the opportunities to contribute to the field. In this regard, we believe that the timely and thorough review of the recent advances in untethered soft actuators will be informative for the general readers who wish to draw insights and gain potential perspectives in the field.
In this article, we introduce the representative works of the untethered soft actuators that serve as breakthroughs in soft robotics and further discuss the imminent challenges of the soft actuators to be addressed. Soft actuators can also be applied to rigid robots since the actuators reviewed in this paper operate in an untethered configuration. However, we intentionally circumscribed the scope and focused mainly on soft actuators for soft robots because the incorporation of soft actuators into the soft robot can make the entire robot soft and compliant. There exist specific applications where the soft robots exhibit comparative strengths over rigid robots such as navigating through the tortuous space16,17, exploring deep-sea at extremely high pressure18, or minimally invasive surgery19. Furthermore, to present these works systematically, the paper categorizes the soft actuators by four representative working mechanisms (1. pneumatically/hydraulically-driven, 2. magnetically-driven, 3. heat-driven, and 4. electrically-driven) and further examines each actuating mechanism in relation to the untethered soft robots as illustrated in Fig. 1. The paper examines the strengths and limitations of each actuating method and concludes with the future perspective of untethered soft actuators for soft robotics. Box 1 provides the general summary that addresses the strategies to provide the power source for actuation control of the soft robots. Additionally, Table 1 draws the overall comparison of each soft actuating method to highlight the strengths, weaknesses, and other key features such as response time and output force range. On the other hand, Table 2 captures key highlights of representative soft actuators that operate based on a variety of mechanisms and thus delivers a more specific comparison.
a pneumatically-driven actuators. b magnetically-driven actuators. c heat-driven actuators. d electrically-driven actuators. e overview of the essential components for untethered soft robots. Pneumatically-driven actuators operate based on the volume change during phase change while magnetically-driven actuators utilize the interaction between magnetic fields and actuators made up of magnetic materials. Heat-driven actuators can be categorized into three types based on energy conversion: heat-responsive, photothermal, and hydrothermal. Electrically-driven actuators undergo mechanical deformation due to the charge polarization of dielectric materials in response to an applied electric field.
Distinguished by their flexibility, deformability, and adaptability, soft robots offer unique advantages over traditional rigid robots. However, soft actuators face significant challenges, particularly regarding power sources and external wiring because soft actuators require effective power sources for actuation to enable movement in soft robots. Recently, various options such as pneumatics, electricity, and magnetics have been explored, but the dependence on external wiring restricts mobility and functionality. Contemporary advancements have introduced soft actuators that eliminate the need for external wiring to enhance the capabilities of soft robots.
For instance, unlike the pneumatic actuators that traditionally rely on bulky pumps, innovations such as pneumatic actuation, based on phase change materials, circumvent this limitation, allowing for untethered operation. Additionally, external stimuli like magnetic fields, heat, and electricity can provide mechanical force, eliminating the need for physical connections and enabling greater mobility.
The article categorizes untethered soft actuators into four mechanisms: pneumatic/hydraulic-driven, magnetic-driven, heat-driven, and electric-driven, evaluating their strengths and limitations. Looking ahead, key challenges must be addressed for the widespread adoption of soft actuators, including reducing fabrication costs, ensuring manufacturing reproducibility, achieving precise force output, and enhancing material durability. Collaboration among researchers, industry, and the development of standardized practices will be crucial for advancing soft robotics and unlocking their potential across various applications.
Pneumatic and hydraulic actuators are capable of generating an enormous magnitude of mechanical force at a high response rate (~ 0.1 s) as they provide energy transmission through the mechanical properties of liquid and air20. For instance, soft pneumatic and hydraulic actuators are widely employed in practical applications such as soft grippers21,22,23,24,25,26, soft haptic devices27, and bioinspired robots9,28,29,30. However, pressurizing the pneumatic and hydraulic fluids requires pumps and fluid chambers that are usually too bulky and heavy to be integrated into the soft robot. Besides, utilizing the pumps and fluid chambers involves the pneumatic/hydraulic tubing to supply fluid to the actuator, undermining the core functionalities of the untethered soft robots. To address these issues, several studies developed soft pneumatic actuators that do not incorporate pumps but rather resorted to liquid-vapor transition to induce the dramatic volume change during the phase change31,32,33. The work in Fig. 2a utilizes a representative pump-less pneumatic actuator that operates based on the liquid-vapor transition to drive locomotion. The soft actuator in this study uses the flexible thermoelectric device to induce the inflation of the pneumatic chamber by liquid-vapor transition and, thus, to mimic the crawling movement of the earthworm34. The thermoelectric device offers desirable properties for pneumatic actuators since it can both cool and heat with a single device structure, suggesting that it can accelerate both liquid-to-vapor and vapor-to-liquid transitions. In addition, the thermoelectric device is lightweight, noise-free, and compact, making it a preferred candidate that worked based on the phase transition for the pump-less actuator. Exploiting the advantages of thermoelectricity, another study also developed a soft fish robot that can explore underwater space with the soft pump-less actuator, as depicted in Fig. 2b. The explosive volume change during the phase transition enables localized buoyancy control, which allows the soft fish robot to move forward and make a turn35. Both the pump-less soft actuators utilize untethered communications for piloting and onboard battery to provide the power source for thermoelectric devices, exhibiting a completely untethered configuration. However, despite the desirable features of the soft pump-less actuator based on the thermoelectric device, these actuators suffer from a relatively low response rate and thereby result in slow movements because phase change usually consumes a substantial amount of thermal energy. Other research groups also developed pneumatic actuators based on the liquid-vapor transition by actively heating (Joule heating) in order to control the buoyancy in the underwater environment33,36. Yet, these pneumatic actuators lack the cooling function to induce the vapor-to-liquid transition to lower the buoyant force by reducing the volume of soft actuators. Also, they showed a slow response rate to produce the buoyant force since the phase transition usually requires a substantial amount of energy. Several studies demonstrated pump-less soft actuators based on phase transition that was induced by the magnetic field. However, they do not show as high a response rate as conventional rigid robots37,38,39. Another way to achieve an untethered soft actuator based on pneumatics or hydraulics is to develop a soft and lightweight electronic pump that can be installed directly on the soft robot body. However, most of the recent studies on the soft pump involve pneumatic tubes or electrical wires40,41,42,43,44,45,46,47, except a few studies48,49,50. A representative study on an untethered soft electronic pump operates in the presence of a strong non-uniform electrical field that induces the movement of electrons and liquid molecules, and the liquid can move in both directions through the soft pump according to the electrical field. To design the soft actuator and robot completely untethered, the authors developed a lightweight miniaturized high-voltage converter that can transform the low-voltage input from the battery to high-voltage output for operating the soft pump. The soft miniature pump in this work can generate high hydraulic pressure and flow rate with a practical response rate (t ≈ 1 s), making it a highly potent candidate as the soft actuator for the soft integrated robot (Fig. 2c)48. Nevertheless, the volume of the fluid chamber can complicate the overall design of the soft robot because the greater the volume of the fluid chamber becomes, the bulkier and heavier the overall system of the soft robot gets. Yet, at the same time, the volume of the fluid chamber is directly proportional to the actuator capacity, so it requires meticulous design consideration to maximize its performance. Also, since the soft robots based on the soft electronic pumps do not support untethered communication for robot piloting, there still exists room for improvement.
a Pumpless thermoelectric pneumatic actuator and soft earthworm robot. Reproduced with permission from ref. 34, copyright 2023, Elsevier BV. b Soft robotic fish based on the thermoelectric pneumatic actuator. Reproduced with permission under CC BY 4.0 license from ref. 35. c Soft electronic pump and robotic fish/vehicle based on the soft electronic pump. Reproduced with permission under CC BY 4.0 license from ref. 48.
In addition, although we classified the soft actuator research studies based on operating mechanisms, there are overlaps between specified categories because one arbitrary energy can be converted into another form to operate the actuation at the end. For instance, light energy can induce the liquid-vapor transition as an input energy such that the soft actuator eventually operates based on the pneumatics. A recent study by Shao et al. proposed the 4D printed, untethered soft actuator that undergoes volume expansion as a result of the liquid-vapor transition of methanol in the presence of the light input. Technically, when the light is illuminated on the actuator, the actuator absorbs light, and the absorbed light is converted to heat, which serves to cause the phase transition of methanol51. Such an actuation method based on light-driven phase transition does not require the onboard battery incorporation to provide power, making the entire structure lightweight and remarkably simple. Nonetheless, multiple energy conversion processes, for instance, from light to heat and then to the phase transition that leads to the volume change, indicate that there might be an energy loss during the energy conversion. The energy losses after a series of energy conversion processes can affect the overall efficiency of the actuation and slow down response time as a result. Thus, since there exists a clear trade-off between the structure complexity and energy efficiency, careful design considerations should be made according to the application of the soft robots.
To summarize, the recent advances in pump-less soft pneumatic and hydraulic actuators have shown great promise since they do not only require any pneumatic tubes connected to pumps and fluid chambers but also, at the same time, produce a sufficient amount of force to be used practically. However, the pump-less actuators rely on the bidirectional phase transition between liquid and gas that requires a considerable amount of thermal energy and thus often results in a poor response time. Alternatively, there have been efforts to develop a lightweight and compact pump for soft robotics such that the incorporation of the pump does not interfere with the overall design of the soft robots. Also, the light-driven pneumatic actuator presented a novel method to cause mechanical deformation based on a simple architecture that does not incorporate the onboard power source such as a battery. Even though the soft electronic pump and light-driven actuator offered immense potential as a future driving force to operate the untethered soft actuator, it has to be logically designed to enhance the overall performance of the soft robot.
Magnet-driven untethered soft actuators, which are actuated by the influence of magnetic fields, draw inspiration from the way magnetic fields guide the behavior and movements of specific organisms. These actuators offer remarkable prospects for a wide range of applications. The advantages of using magnetic fields as an untethered external stimulus for actuating soft materials are distinct and multifaceted: remote controllability, high spatial precision, and adaptability across diverse environments. Magnetic fields have the unique ability to penetrate numerous materials, have freely generatable spatial gradients, and can be easily decoupled from other stimuli52,53,54,55. This makes them particularly versatile in shaping and controlling actuator behavior in response to external conditions. Moreover, a response of the material to changes in magnetic fields tends to be comparatively swift, rendering magnetic actuation an effective method for responsive and adaptive soft devices56,57,58. These advantages have shown great application potential for untethered soft actuators and robots such as bio-inspired designs50,59,60,61,62,63,64,65,66,67, magnetically-responsive materials15,68,69,70,71,72,73,74,75, micro/nanoscale actuators and robot62,76,77,78,79,80,81, and dynamic/reconfigurable structures66,82,83,84,85,86,87,88.
Bio-inspired designs and dynamic, reconfigurable structures form the foundation for progress in the field of untethered magnetic soft actuators. Emulating the intricate movements of organisms, a group of researchers has created bio-inspired robots that mimic the locomotion of earthworms and squids (Fig. 3a). Their design utilizes a diaphragm formed from a single mold that is radially magnetized, which allows for significant two-directional and 3D deformations when exposed to a low homogeneous magnetic field50. Moreover, another work presented a type of miniature, bio-inspired soft electromagnetic robots built from curved bilayers of elastic material, propelled by the influence of Lorentz forces. The authors incorporated channels filled with printed liquid metal that transmit alternating currents, which is capable of versatile locomotion, including walking, running, swimming, jumping, steering, and cargo transportation at high speeds, as depicted in Fig. 3b67.
a Bio-inspired magnetically-driven diaphragm and the soft robot based on the diaphragm actuator. Reproduced with permission under CC BY 4.0 license from ref. 50. b Small-scale soft electromagnetic robot made up of elastomeric bilayers. Reproduced with permission under CC BY 4.0 license from ref. 67. c Untethered small-scale magnetic soft robot with programmable magnetization. Reproduced with permission under CC BY-NC 4.0 license from ref. 93, copyright 2022, AAAS. d Untethered magnetic soft actuators based on liquid crystalline elastomers. Reproduced with permission under CC BY-NC 4.0 license from ref. 87, copyright 2022, AAAS.
However, these advanced designs face substantial challenges since replicating bio-inspired movements solely is done by the modulation of magnetic fields. Unlike organisms that rely on a myriad of motor proteins and mechanical structures, magnetically driven actuators are limited to manipulating magnetic fields. Addressing these challenges, researchers focused on developing autonomous decision-making capabilities of bio-inspired magnetic soft robots, enabling them to intelligently respond to changes in magnetic fields, just as how biological organisms adapt their behavior based on sensory inputs89,90,91,92. A representative work in Fig. 3c utilized programmed magnetization patterns in soft robots, offering new possibilities for application related to multimodal robot locomotion with environmental sensing and detection93. In another research, the proposed fabrication strategy transforms 2D magnetic sheets into 3D soft magneto-active machines, enabling high-throughput production and various applications, including untethered biomedical robots, electronic robots, and mechanical encoders93. Still, inherent limitations in magnetic field control for complex three-dimensional movements present further difficulties, particularly over longer distances55,94. To address these challenges, researchers developed dynamic, reconfigurable, and magnetically responsive structures that can magnetically actuate soft materials with versatile contraction-derived motions and local magnetic control, as illustrated in Fig. 3d. Their work highlights the difficulties in achieving local and sequential magnetic control using multiple magnetization thresholds87.
Taken together, these exemplary works underline the recurring theme of challenges related to magnetic field manipulation. To overcome these hurdles and realize the full potential of magnetic soft robots, significant advancements in materials science and magnetic field control techniques are needed, thereby paving the way for the next generation of autonomous magnetic soft robots.
Heat-driven actuators, an essential part of untethered soft robotics, possess unique characteristics and advantages since heat-driven actuators convert readily available thermal energy directly into mechanical work11,95. There exist several types of heat-driven soft actuators since energy in the other forms can be converted to heat that serves to induce the deformation of the actuators. Indeed, the most intuitive type would correspond to the actuators that respond to temperature change or heat itself (heat-responsive actuators). On the contrary, the actuators can also absorb light, which is converted into heat and serves to change the temperature of the specific actuator region, such that the actuators operate in the presence of light (photothermal actuators). Another type of actuator would be a hydrothermal actuator because the humidity or moisture is closely associated with temperature. Thus, this section will examine several types of representative heat-driven soft actuators in subsequent subsections.
Heat-responsive soft actuators undergo mechanical deformation in the presence of temperature change or heat exchange with the external environment. Heat-responsive actuators are primarily made of materials that are capable of undergoing considerable deformations to generate high force outputs when heated96,97,98, and these include materials such as liquid crystal elastomers (LCE)s99,100,101,102,103,104, hydrogel105,106, and shape-memory polymers (SMPs)107,108,109,110,111,112. One study demonstrated a 4D-printed untethered robot composed of LCE, which self-propelled when heated above 160 °C, illustrating the remarkable potential of heat-driven actuation100 (Fig. 4a). Twisted-and-coiled actuators (TCAs) hold promise for powering centimeter-scale soft robots due to their cost-effectiveness, high work density, and electrical operability, although their elevated temperature requirement may limit certain applications. Another research introduced an enhanced TCA fabrication method, enabling 48% free stroke capability and versatile motions in compact soft robots, with the potential for untethered operation using onboard electrical components113. Nonetheless, the elevated temperature threshold may limit its usage in certain applications, as it could lead to safety concerns or thermal damage to surrounding components. On another note, the relatively slow response time of heat-driven actuators can restrict their use in applications requiring rapid and high-frequency operation114,115. However, the relatively slow response time of heat-driven actuators can restrict their use in applications requiring rapid and high-frequency operation114,115. A notable work addressed this limitation by creating strain-programmable artificial muscles that demonstrated thermal and optical controllability, resilience across numerous deformation cycles, and a lifting capacity of >650 times their weight, as depicted in Fig. 4b116. While this work represents a significant advancement, improving the actuation speed without compromising the force output serves as an ongoing challenge in this field. Also, heat-responsive actuators often employ versatile materials that can be molded into diverse shapes and configurations to provide design flexibility and possible cost reductions. A representative study demonstrated the fabrication of active hinges using LCE bilayers and achieved significant reversible bending responses to thermal stimuli, resulting in self-assembling and self-propelling “rollbots“102 (Fig. 4c). Yet challenges persist in ensuring consistent response times and careful synchronization when employing multiple active hinges in a single system. Additionally, thermal-responsive poly(isopropylacrylamide-co-2-(dimethylamino)ethyl methacrylate)/alginate hydrogels have been developed, showcasing the adaptability of heat-responsive materials for intricate shape-changing behaviors and versatile application106. Researchers also explored new possibilities by combining electrical and thermal inputs to achieve complex motions with the ionic polymer-metal composite actuator, representing a significant breakthrough in controlling twisting and bending deformations117.
a 4D-printed untethered soft robot with tactile perception. Reproduced with permission from ref. 100, copyright 2021, Cell Press. b Artificial muscle soft actuator based on strain programmable fibers. Reproduced with permission from ref. 116, copyright 2019, The American Association for the Advancement of Science. c Untethered soft actuator with passive control of shape morphing. Reproduced with permission from ref. 102, copyright 2019. The American Association for the Advancement of Science. d Programmable heat-driven actuator and soft crawling robot. Reproduced with permission under CC BY 4.0 license from ref. 101.
The sensitivity of heat-responsive actuators to environmental temperature also remains a notable challenge because unintentional actuation can occur due to ambient temperature fluctuations that might lead to unintended movements and reduced accuracy118,119. In an attempt to address this issue, a group of researchers used a caterpillar-inspired robot design and introduced a patterned soft heater to an LCE-based thermal bimorph actuator (Fig. 4d). This design utilized programmable heating to achieve different temperature and curvature distributions, enabling bidirectional locomotion and better control101. Another example of a bioinspired robot design using thermally responsive hydrogels like poly(N-isopropylacrylamide) (PNIPAM) was demonstrated, enabling complex shape changes, including an artificial flower that blossoms and reverses with temperature shifts105. Despite the advancements, the fine-tuning of temperature control and heat distribution still presents a significant hurdle, especially when scaling to larger systems.
In summary, heat-responsive actuators offer several advantages, including manufacturing simplicity and high-force output, making them attractive for various soft robotics applications. These benefits stem from their ability to convert thermal energy into mechanical work, which can lead to reduced energy consumption, passive actuation capabilities, and sustainability in certain scenarios. However, it is crucial to acknowledge the challenges these actuators face, such as environmental sensitivity, response time limitations, and safety concerns in high-temperature operations. For example, their performance may be affected by ambient temperature fluctuations, potentially resulting in unintended movements or reduced precision. Achieving optimal energy efficiency often requires precise temperature control, which can be challenging, especially when scaling up or operating in dynamic environments. Researchers are actively working to enhance the overall performance of heat-responsive actuators, aiming to maximize their benefits while mitigating limitations.
Natural species have evolved to thrive in specific environmental lighting conditions, often evolving their mobility responses to light, as seen in sun-tracking plants and photoreceptive muscle fibers. Drawing inspiration from these biological mechanisms, numerous studies have extensively employed light as a untethered external photothermal stimulus source to drive the motion of soft actuators in the past decades120,121,122,123,124. Light offers clear advantages as an external stimulus source for soft actuators due to its ability to facilitate the motion control of soft actuators in rapid response, high precision, and non-invasive manners, which is achieved by modulating various light parameters, including wavelength, intensity, and polarization to leverage diverse operating mechanisms.
An illustrative example of harnessing the photothermal effect can be observed in Fig. 5a, where a dandelion-inspired light-driven actuator was developed. This actuator employed gold nanorods to achieve selective optical absorption and high photothermal conversion efficiency, aiming to mimic the motion of insect-scale micro aerial vehicles125. The dandelion-inspired actuator, characterized by its tubular shape, addresses the issue of randomly-flighting motion typically associated with conventional artificial dandelion devices. Consequently, it allowed for controlled rotation in both clockwise and counterclockwise directions. Additionally, liquid crystal elastomers (LCEs) have also been widely utilized for photosensitive actuators. The micro-scale fiber actuator was developed through the utilization of an electrospinning technique applied to LCEs (Fig. 5b), exhibiting high actuation strain (~ 60%) with a rapid response time (<0.2 s)126. This innovative approach effectively addressed an issue associated with macro-scale LCE fibers (> 0.3 mm), characterized by slow actuation response. The results presented exceptional actuating performance comparable with that of human muscle fibers.
a Artificial microflier inspired by dandelions and their components. Reproduced with permission under CC BY 4.0 licence from ref. 125. b Laser-driven soft actuator based on electrospun LCE microfiber coated with PDA. Reproduced with permission from ref. 126, copyright 2019, The American Association for the Advancement of Science. c Light-initiated actuation of an LCE catapult with tunable and programmable snapping dynamics. Reproduced with permission from ref. 127, copyright 2023, Wiley.
Moreover, to enhance the performance of a photothermally-driven soft actuator, the interesting work is presented in Fig. 5c127. Inspired by the snapping motions observed in various living organisms, the researchers utilized a reconfigurable structural constraint into a photosensitive LCE actuator that mimics the snapping motions observed in various living organisms. The novel approach resulted in an actuator with an exceptional rapid motion (up to 2.5 m s−1), achieved by effectively converting elastic energy to kinetic energy, implying that the incorporation of reconfigurable structure into soft actuating systems holds promise for the development of ultrafast soft robotics. Furthermore, to address the challenge of soft material with inherently weak mechanical properties, a group of researchers introduced nanofillers into polymer matrices. This approach was inspired by the human-muscle fibrous system128. Benefitting from the exceptional thermal and mechanical properties of graphene fillers, the resulting artificial muscle fibers displayed not only rapid actuation upon exposure to near-infrared (NIR) irradiation but also remarkable power density (293 W kg−1), which is about 6 times higher than that of human muscle. Notably, the reversible filler percolation network configuration effectively mitigates the inherent mechanical weakening of fiber-based soft actuators in the contracted state.
In summary, many researchers have focused on the diverse photothermally-driven soft actuators. However, there remains a need for future studies to address critical challenges associated with conventional approaches. For instance, for the microscale photothermally-driven actuator such as the microflier, a crucial consideration should be the miniaturization of electronic components to enable seamless integration into small-size actuators, thereby enhancing programmable controllability. Moreover, to achieve the rapid response for the fiber-based photothermal actuator, an effort should focus on reducing fiber diameter (<10 μm) because the larger fiber diameter results in a slow thermo-actuation response, as aforementioned. Besides, as the diameters of LCE fibers have been reported to greatly deviate (10 ~ 100 μm), it is of great importance to achieve high uniformity of fiber diameters for practical applications such as microfiber robots.
In nature, humidity plays a significant role in influencing the behavior of living organisms through swelling or deswelling induced by water129. Moreover, it is worth noting that humidity and moisture levels are greatly affected by temperature variations. In this section, we demonstrate a soft actuator that responds not only to changes in humidity but also to solvent evaporation triggered by thermal stimuli. Among the various natural objects, pine cones are one of the representatives that exhibit deformation in response to different humidity conditions130,131. This hygroscopic behavior in pine cones is intricately linked to their ability to open or close in response to environmental humidity variations, driven by changes in the moisture content within the cones. This behavior originates from the substantial divergence in hygroscopic expansion coefficient among different tissues within the pine cone. Based on the simple mechanism, pine cone-inspired soft actuators have been widely reported in recent years132,133.
Nevertheless, there exists an urgent need to further explore the intricate bending mechanisms of the pine cones, given the relative lack of research into the remarkably slow deformation process observed in pine cones as compared to other hygroscopic plants. In this regard, a group of researchers revealed that the ultra-slowly hygroscopic deformation of the pine cones is mainly attributed to the distinct vascular bundle structures characterized by spring/square microtube configurations134. By further exploring the hygroscopic behavior, which operates at an ultra-low speed and is primarily governed by the unique heterostructures, the researchers developed soft actuators. These soft actuators, integrated with the heterostructure through material design, facilitate imperceptible yet controllable motions, thereby offering valuable insights into the various fields where imperceptibility is crucial, including camouflage and reconnaissance. Moreover, Aziz et al. developed a plant-like artificial muscle at the micro level by imitating the helical plants in nature (Fig. 6a)135. The hierarchically chiral microstructures enable plant-like actuators to deform with a large stroke and rapid velocities, akin to natural plants that exhibit hydrotropism or thermotropism through hydrothermal volume expansion and contraction process induced by changes in humidity or heat.
a Plant-inspired soft artificial muscles with tropism mechanism. Actual photograph of a live plant tendril (left) and graphical representation of plant-like actuator with macro-/micro components. Reproduced with permission from ref. 135, copyright 2023, Wiley. b Natural pollen paper-based humidity-driven soft actuator. Reproduced under CC BY-NC 4.0 license from ref. 136, copyright 2020, NAS. c Programmable actuation by direct pen writing. Reproduced with permission from ref. 137, copyright 2023, Wiley. d Light motor based on hydrothermal solvent evaporation. Reproduced with permission under CC BY 4.0 license from ref. 138.
As green electronics have extensively attracted significant attention in a variety of fields, environmentally sustainable actuators have been extensively explored. Researchers have developed eco-friendly paper-based actuators derived from naturally abundant pollen grains (Fig. 6b)136. The actuator exhibited asymmetric microstructure on the top and bottom surfaces induced by water evaporation during the fabrication process. This asymmetry resulted in distinct deformation degrees of top and bottom surfaces, enabling locomotion under different humidity conditions. The performance of the paper-based actuator can be fine-tuned by controlling the paper thickness and the fabrication process. Furthermore, Yang et al. developed a humidity-sensitive synthetic earthworm as a degradable actuator capable of emulating the behavior of natural earthworms. The unique ability to function in soil is facilitated by the capacity of water to penetrate through the soil137. Especially, the employment of pen-writing patterning technology into a bilayer film composed of hydrophobic/philic layers results in programmable actuation with more precise control in the desired ways, thereby enabling the actuator to operate as intelligent soft robots to mimic the behavior of natural worms (Fig. 6c).
The direct conversion of light energy into mechanical energy has attracted significant attention due to small efficiency losses, particularly when compared to conventional energy conversion methods such as photovoltaics, which involve multiple energy conversion steps and may result in energy losses. The research presented in Fig. 6d introduces a light-driven oscillator that operates based on a porous structure designed for rapid adsorption and desorption138. The solar engine-like oscillator exhibited a maximum power of (15.4 × 10−2 W kg−1) and can ceaselessly operate under solvent supply. Thus, the oscillator holds significant promise for practical applications such as use in universe exploration and rescue operations in challenging, remote areas that are difficult for human beings. Its capacity to respond to diffuse light sources such as sunlight distinguishes it from the previous oscillators, which were primarily activated by tightly focused incident light, typically generated by laser beams.
Recently, the exploration of natural organisms as bionic models for hydrothermally-driven soft actuators has garnered considerable attention139,140. Likewise, hydrothermally-driven untethered soft actuators, which can operate under non-invasive conditions unlike other types of actuators driven by high electrical input, high temperature, and UV light, have attracted significant interest from a variety of researchers, thereby extensively employable in biomedical applications141. Nevertheless, for practical, real-world applications, there is a pressing requirement to develop highly sensitive hydrothermal-based actuators. These actuators must reliably function even within a subtle variation in environmental humidity, especially based on a deeper understanding of the operational mechanisms, not just shape-mimicking actuators.
The electrically-driven soft actuators are the type of actuators that operate with the electrical input. The use of dielectric elastomer actuators in Fig. 7 exemplifies this since these actuators produce mechanical deformation in the presence of voltage application. Electrically-driven soft actuators present significant benefits in untethered soft robotics, including versatile actuation mechanisms, mechanical flexibility, swift response times, and adaptability to a multitude of environments95. Various types of electrically-driven soft actuators have emerged, harnessing materials like liquid metals, shape memory alloys, conducting polymers, and hydrogels. These actuators offer versatile capabilities, with electronic signals facilitating precise control of their motion characteristics, and they can be seamlessly integrated with electronic devices and drivers, making them invaluable in fields like microfluidic systems142,143, manipulation of micro/microscale object18,144,145, and bio-inspired microrobots146,147,148. In one exemplary work, an origami-inspired approach integrated sensing, computing, and actuation directly into pliable and conductive materials, thus developing an autonomous robot with the capacity to interact dynamically with its environment (Fig. 7a)146. This integrated approach not only enhances the autonomy of the robots but also leverages the advantages of origami-based fabrication, offering multiple functionalities and a versatile platform for future innovations. In many cases, such actuators utilize electroactive polymers or dielectric elastomers that can generate substantial force output and potentially operate at higher frequencies149. Also, other researchers allowed the creation of a subgram, insect-sized robot capable of autonomously navigating and carrying payloads five times its weight using low-voltage stacked dielectric elastomer actuators (LVSDEAs) operating below 450 volts, showcasing the potential for resilient and fast untethered robots in soft robotics research144. Additionally, a representative example in Fig. 7b shows the development of self-contained soft electrofluidic actuators (SEFAs) that are fabricated based on basic techniques with widely accessible materials, demonstrating exceptional safety, reliability, controllability, durability, versatility, and swift response142. However, technical challenges remain in the high operating voltages due to the use of dielectric liquid along with the risk of dielectric breakdown if the actuator undergoes excessive strain150,151.
a Origami-based robot with autonomous functionality. Reproduced with permission under CC BY 4.0 license from ref. 146. b Untethered powered Untethered soft electrofluidic actuator that uses electrically responsive fluids. Reproduced with permission under CC BY-NC 4.0 license from ref. 142, copyright 2021, AAAS. c Bio-inspired snailfish-like untethered soft underwater robot. Reproduced with permission from ref. 18, copyright 2021, Springer Nature. d Dexterous electrically-driven soft robots with reconfigurable design. Reproduced with permission under CC BY 4.0 license from ref. 154.
Addressing the need for environmental adaptability152,153, a group of engineers achieved a significant breakthrough with the design of an untethered and self-powered, electrically-driven soft robot fit for deep-sea exploration as illustrated in Fig. 7c18. This distinctive robot employs electrically-driven actuation to traverse deep-sea environments, safeguarding its integral electronics against extreme pressure by encapsulating them within a silicone matrix. Likewise, another research concentrated on enhancing soft robot mobility through a chiral-lattice design method, allowing immediate direction changes and complex motions controlled by voltage frequency (Fig. 7d)154. This approach marks a significant leap toward versatile, autonomous soft robots, capable of navigating complex environments and executing diverse tasks with remarkable agility and precision. Furthermore, the researchers developed a manta ray-inspired soft electronic fish using a dielectric elastomer actuator, with safety and stability enhanced by utilizing encapsulated hydrogel and surrounding tap water as an electric ground148.
In conclusion, electrically-driven actuators offer significant advantages, including quick response times and the potential for high-force output, making them well-suited for a wide range of applications. These actuators leverage principles like electromagnetic actuation and piezoelectric materials, allowing them to generate substantial mechanical forces. Their capability for high-force output is particularly valuable in scenarios where robust mechanical power is essential, such as robotics and automation. However, it is important to note that the exact force levels achievable with electrically driven actuators can vary depending on factors like their design, materials, and the specific application context. While they have the potential for high-force output, the actual force generated may need to be optimized to meet the unique demands of each application.
Furthermore, while electrically driven actuators are generally less sensitive to factors like temperature, light, and moisture when compared to some other types of soft actuators, they can exhibit sensitivity to factors like electrical interference, electromagnetic fields, and power supply stability. These considerations are crucial for ensuring their reliable performance in real-world applications. Additionally, extreme environmental conditions, such as exposure to moisture or high humidity, can impact the electrical components and safety of electrically driven actuators. Therefore, careful design and environmental management are essential to maximize their effectiveness and safety. In summary, electrically driven actuators have the potential to deliver high-force output, but the actual force levels may vary based on design and application. Their performance advantages must be balanced with considerations of environmental sensitivity and other application-specific factors to ensure their successful deployment in diverse environments and applications.
Based on a variety of soft actuators, as we discussed, to develop fully soft robots with untethered operation systems, a myriad of research has been devoted to integrating the various soft actuators with functional components such as sensors and powering sources18,38,155. For instance, as aforementioned, a group of researchers developed an untethered soft robotic fish by incorporating a printed circuit board and battery module35. This exemplifies a typical approach in which soft actuators are integrated into soft robotic systems alongside conventional functional components such as circuit boards, power sources, and microelectronics, which are rigid and bulky34. Nevertheless, this approach poses several challenges, including the potential for mechanical mismatch arising from the integration of rigid components into soft materials. Furthermore, there are concerns related to the potential scaling issues of soft robotic systems to accommodate a diverse array of functional components. These limitations necessitate further investigation and careful design considerations in order to effectively address their implications.
To address these challenges, extensive research has focused on developing soft sensors156, energy storage157, and energy harvesters158 to replace bulky components in recent decades, thereby establishing fully soft robots with untethered operation systems. Nevertheless, the critical issues still remain challenging, including the complex integration process and the delicate issue of mechanical mismatch. One viable solution is to achieve a unified soft robotic system by seamlessly integrating functional capabilities during the manufacturing stage159,160,161. For instance, Dong et al. provided seamlessly multilayered coaxial fibers that can self-sense and actuate as all-in-one artificial fibers162. Moreover, a smart humidity-responsive robot recently demonstrated remarkable intelligence in object manipulation and perception, exhibiting excellent stability across a broad range of relative humidity levels (10 ~ 75%)163. This robot autonomously generates distinct electrical signals corresponding to the type of objects that it interacts with. This functionality is accomplished through the triboelectric effect, initiated by contact between the objects and the gripper, which functions as a soft gripper. Consequently, the smart robot achieves simultaneous actuation and perception without the need for additional integrated components, advancing the development of lightweight and intelligent robotic systems.
Furthermore, for active control of systems, another research group devised a feedback control platform designed to maintain a target temperature without the need for a dedicated temperature sensor. This achievement is made possible by leveraging the temperature-dependent resistance of a nanowire heater, which is seamlessly integrated into the robotic systems, serving both as a heat source and an indirect parameter for temperature estimation164. These considerable endeavors have been directed toward reducing the complexity associated with robotic systems, thereby leading to the advancement of smart soft robotics.
Likewise, in the endeavor to achieve fully intelligent soft robotics with untethered operation systems, inherent challenges arise within the realm of sensing part. These issues encompass nonlinear output and hysteresis165,166,167,168, which not only complicate integration but also pose significant challenges in achieving proprioception among soft actuators. Moreover, the limitations associated with relying on a single sensor prevent complete self-sensing capabilities. To combat these challenges, significant efforts have been dedicated to leveraging machine-learning techniques as a promising solution169. For example, Shu et al. developed proprioceptive electronic skins enhanced by machine learning technology. These integrated systems endowed soft robots with outstanding capabilities to perceive and adapt to their surrounding environment, which implies their potential for practical, real-world applications170.
The recent advances in soft actuators have bestowed the soft robots with fascinating functionalities as soft actuators are responsible for generating mechanical force, and especially the new type of untethered soft actuators have a promising potential to be applied in various fields such as autonomous robotics, smart haptic devices, and untethered medical robots. We believe that the future perspective of soft actuators and robots is clear: they should find the applications, to which the traditional robots can not be applied due to their rigid bulky structures. In the previous sections, we have thoroughly reviewed several types of untethered soft actuators that were published recently, and untethered soft actuators of different kinds have suitable applications of their own. For instance, it will be quite challenging to apply pneumatic and hydraulic soft actuators for drug delivery or invasive surgery in the form of a millimeter robot since pneumatic and hydraulic soft actuators require fluid chambers that take up a substantial volume. Instead, it is more suitable for pneumatic or hydraulic soft actuators to be applied to autonomous robotics34,35,48,171, haptic devices49, or assistance in medical procedures172,173,174,175,176 because they can generate a greater amount of force when compared to other untethered soft actuators. Especially for medical rehabilitation therapies, soft robotic exoskeletons or exosuits based on untethered pneumatic actuators can provide enough force to support and assist to individuals with mobility impairments or help with rehabilitation therapies, as opposed to other types of untethered actuators. For drug delivery or surgical applications, untethered magnet-driven soft actuators have desirable properties as their movements and configuration can be controlled accurately from outside by the magnetic field177,178.
Furthermore, as opposed to pneumatic and hydraulic soft actuators that demand artificial input, some of the external stimulus-driven actuators receive input from the natural surroundings, and such naturally occurring inputs have profound implications for the soft actuators as they can be easily associated with physical intelligence. Physical intelligence is another field of study that receives much attention from soft robotics since it does not incorporate artificial intelligence or human input for its movements. Physical intelligence can be defined as the physical encoding of an agent’s sensing, actuation, control, memory, logic, computing, adaptability, learning, and decision-making capabilities179, so it requires a collective integration of interdisciplinary studies to encode physical intelligence into the thoroughly designed soft robot since it requires the careful selection of materials and structural design for both sensing and soft actuator to enhance the maximized functionalities of the soft robot. As discussed previously, several types of robots that are driven by naturally occurring external stimuli such as heat180,181, light182,183,184,185, humidity186,187,188,189, or even multiple stimuli190, fall into this category. Physical intelligence offers novel functionalities for untethered external stimulus-driven soft actuators because soft actuators can produce cyclic movements even in the absence of artificial inputs or human intervention, making them highly autonomous. Furthermore, soft robots with physical intelligence are capable of sensing and actuating with the single device structure, suggesting that they do not need to incorporate additional onboard circuitry for embedded artificial intelligence and sensors that might interfere with the general movement of the soft robots.
Despite the fascinating properties and feasible applications of untethered soft actuators, there exist several challenges that soft actuators need to address. The most significant challenge of soft actuators would arise from a seamless integration of core components into a functional untethered soft robot. For instance, even if the arbitrary soft actuator is capable of generating a considerable amount of mechanical force, the ideal type of soft robot consists of various sensors, embedded artificial intelligence, and a power source, which usually take the physical form of the printed circuit board (PCB) and battery. Therefore, the incorporation of circuitry and battery components might add unwanted weight and volume that might degrade and even conflict with the performance of the soft actuator.
Along with the added components that might impair the actuating efficiency, soft actuators often lack built-in sensing capabilities, making it challenging to obtain real-time feedback on their deformation and force output. Despite remarkable technological advances in soft sensors, soft sensors still lack reliability and accuracy when compared to rigid commercial sensors, and thus integrating sensors into soft actuators or developing external sensing mechanisms to provide feedback for closed-loop control can be a complex task. Also, the lack of accuracy and reliability of soft sensors can complicate the robotic control and feedback mechanism since soft actuators often exhibit non-linear and complex behavior191, making their control and precise manipulation challenging. In this regard, developing control strategies and feedback mechanisms that can accurately regulate the movement and deformation of soft actuators is crucial for achieving desired robot behaviors.
Another challenge the soft actuators need to overcome is modulus mismatching with rigid materials. Since Young’s modulus of soft robots matches that of the human skin, the human-machine interfaces serve as one of the most promising applications of soft robots. The soft and flexible nature of soft robots makes them more comfortable and safer to interact with humans. Nevertheless, there exists a demand in which both soft and traditional robotics are employed in a hybrid form. In this case, modulus mismatching can become a critical factor that affects the general performance since it can affect force transmission and control. Modulus mismatching can influence the transmission of forces from the actuator to the end effector of the soft robot or the object it interacts with. Thus, optimization of the stiffness of the actuator relative to other components should be carried out carefully such that the robot can transmit forces efficiently and precisely, enabling accurate manipulation or locomotion.
Besides, the durability and reliability due to soft and compliant material makeup can cause considerable issues for soft actuators. Soft actuators, especially those made of elastomeric materials, are vulnerable to fatigue and wear over time, and such a change in the mechanical property due to repeated use can affect the performance of soft actuators. Ensuring the durability and long lifespan of soft actuators is important for practical applications, as they need to withstand repetitive movements and deformations without significant degradation in performance.
Despite the incredible advances in soft actuators and their applications in robotics as discussed in this article, the research scope and the applications of the soft actuators still pertain to the academic fields. Rather, for soft actuators to find their true meaning and significance, they need to be utilized in the industry or deployed in the real-life field to deliver their purposes. As discussed throughout this article, soft actuators possess the physical properties and other pertinent features that traditional rigid actuators cannot emulate, so certain real-life applications surely exist that only soft actuators can deliver. For instance, for wearable exoskeletons or movement-assistive devices, the use of soft actuators can reduce the chance of injury or damage due to their soft and flexible nature. To a certain extent, soft actuators show definite advantages when interacting with human interaction or delicate and mechanically fragile objects. Furthermore, if the entire robot is comprised of soft materials, it would allow the soft robot to be deployed to travel through the unstructured and complex environment or even to a deep sea where pressure is extremely elevated – a task that the traditional rigid robots cannot deliver. Nevertheless, the following issues should be fully addressed for the industry commercialization of soft actuators in the overall perspective of soft robotics.
Apart from market readiness and limited awareness of soft robotics, the authors believe that the hurdle mainly arises from fabrication costs. Currently, the fabrication of soft actuators and the entire integration into soft robotic systems are conducted on the laboratory scale. Thus, the cost to manufacture the soft actuator and assemble core components to make a soft robot remains considerably higher than that to manufacture a rigid robot. Nevertheless, the promising realm of 4D printing, an innovative fabrication technique, presents unique advantages and features that hold the potential to mitigate these challenges192. Diverging from conventional 3D printing, 4D printing integrates materials capable of dynamic transformations in response to external stimuli, such as temperature, humidity, or environmental cues. This exceptional capability empowers the creation of intricate, self-assembling structures and sophisticated soft actuators endowed with programmable behaviors193. Despite the emergence of scalable fabrication approaches in the realm of soft robotics, including the compelling avenue of 4D printing, these methodologies have not yet achieved widespread acceptance. This lag in adoption primarily stems from the absence of standardized practices. Since the concept of soft robotics was newly coined in 2012194, the field lacks standardization in terms of materials, design, and control systems, which makes it difficult for the industry to adapt soft robots into their workflow. Moreover, the fabrication recipes. Therefore, the collective efforts from academia and industry are required to reduce and further introduce cost-effective soft robots.
Another issue in the manufacturing side of soft actuators and system integration originates from manufacturing reproducibility and consistent force output. For widespread use and industrial commercialization of soft robots, large-scale manufacturing reproducibility must be guaranteed since the physical properties of the soft materials might be altered even with a minute change in the environmental condition. For instance, humidity and manufacturing temperature can affect the hydrogel-based soft actuators as these factors contribute to variations in the moisture contents inside the hydrogels. Also, the chemical composition of chemical precursors to make finalized materials might vary from batch to batch when it comes to large-scale processing, affecting manufacturing reproducibility in general. The deviation of manufacturing reproducibility can result in inconsistent force/torque output in soft actuators, and this can affect the overall performance and reliability of soft robots. Fortunately, the significant advances in automated technologies that are controlled at a highly precise level should be able to address the manufacturing reproducibility of soft robots in the near future.
One of the main future works that soft actuators have to attain is to generate consistent and precise force output in the presence of the programmed input. Although structural design and material selection can contribute to achieving precise force control to a certain extent, soft actuators cannot produce as precise and consistent force output as traditional rigid actuators due to their inherently compliant material composition. As discussed earlier, this can pose a serious problem since it can eventually lead to reliability issues despite all the favorable properties of the soft actuators. Nevertheless, there are several means to improve the consistency and preciseness of the soft actuators. For instance, due to the rapid developments of soft sensors in a variety of types, the soft sensors can be integrated with the soft actuators to establish the sensory-haptic control feedback such that the force output can be adjusted in real-time. Another way to address this issue is to incorporate machine learning into the soft robot for precise force generation after training. In this regard, although soft actuators alone possess comparative weaknesses over rigid actuators in precise force generation, the system integration with the control algorithm can compensate for the core limitation of the soft actuators.
Due to the compliant and deformable material nature of soft actuators, material durability, including mechanical wear and tear over time as a result of fatigue stress with the industrial setting of high-frequency usage, remains the essential issue to be addressed for the widespread usage of soft actuators in the imminent future because it can definitely affect the performance of the soft actuators. For instance, it can affect the actuation range, precision, force output, response time, and even failure mode. Even though the development of soft yet robust materials can be a direct and definite answer to the fatigue durability issue, roboticists should also invest their efforts to optimize the design of the actuator to minimize the stress concentration and reduce the strain on critical regions.
Addressing the challenges of soft actuators requires interdisciplinary research efforts combining materials science, robotics, control engineering, and manufacturing technologies. In other words, it implies that there still exist plenty of opportunities for soft roboticists to contribute to developing soft actuators further to their practical commercialization. Continued advancements in these areas will contribute to the development of more capable and robust soft actuators, enabling the broader adoption of soft robots in various applications.
Hawkes, E. W., Blumenschein, L. H., Greer, J. D. & Okamura, A. M. A soft robot that navigates its environment through growth. Sci. Robot. 2, eaan3028 (2017).
Justus, K. B. et al. A biosensing soft robot: autonomous parsing of chemical signals through integrated organic and inorganic interfaces. Sci. Robot. 4, eaax0765 (2019).
Hao, Y., Gao, J., Lv, Y. & Liu, J. Low melting point alloys enabled stiffness tunable advanced materials. Adv. Funct. Mater. 32, 2201942 (2022).
Gao, M., Meng, Y., Shen, C. & Pei, Q. Stiffness variable polymers comprising phase‐changing side‐chains: material syntheses and application explorations. Adv. Mater. 34, 2109798 (2022).
Tetsuka, H., Pirrami, L., Wang, T., Demarchi, D. & Shin, S. R. Wirelessly powered 3D printed hierarchical biohybrid robots with multiscale mechanical properties. Adv. Funct. Mater. 32, 2202674 (2022).
Article CAS PubMed PubMed Central Google Scholar
Nardekar, S. S. & Kim, S. J. Untethered magnetic soft robot with ultra‐flexible wirelessly rechargeable micro‐supercapacitor as an oboard power source. Adv. Sci. 10, 2303918 (2023).
Li, Y. et al. Multi‐degree‐of‐freedom robots powered and controlled by microwaves. Adv. Sci. 9, 2203305 (2022).
Iyer , V. , Najafi , A. , James , J. , Fuller , S. & Gollakota , S. Wireless steerable vision for live insects and insect-scale robots .Sci.Robot.5, eabb0839 (2020).
Yang, H. et al. Multifunctional metallic backbones for origami robotics with strain sensing and wireless communication capabilities. Sci. Robot. 4, eaax7020 (2019).
Ozaki, T., Ohta, N., Jimbo, T. & Hamaguchi, K. A wireless radiofrequency-powered insect-scale flapping-wing aerial vehicle. Nat. Electron. 4, 845–852 (2021).
Li, M., Pal, A., Aghakhani, A., Pena-Francesch, A. & Sitti, M. Soft actuators for real-world applications. Nat. Rev. Mater. 7, 235–249 (2022).
Article ADS CAS PubMed Google Scholar
El-Atab, N. et al. Soft actuators for soft robotic applications: a review. Adv. Intell. Syst. 2, 2000128 (2020).
Guo, Y., Liu, L., Liu, Y. & Leng, J. Review of dielectric elastomer actuators and their applications in soft robots. Adv. Intell. Syst. 3, 2000282 (2021).
Rich, S. I., Wood, R. J. & Majidi, C. Untethered soft robotics. Nat. Electron. 1, 102–112 (2018).
Kim, H. et al. Shape morphing smart 3D actuator materials for micro soft robot. Mater. Today 41, 243–269 (2020).
Zhao, Y. et al. Physically intelligent autonomous soft robotic maze escaper. Sci. Adv. 9, eadi3254 (2023).
Article PubMed PubMed Central Google Scholar
Ng, C. S. X. et al. Locomotion of miniature soft robots. Adv. Mater. 33, 2003558 (2021).
Li, G. et al. Self-powered soft robot in the mariana trench. Nature 591, 66–71 (2021).
Article ADS CAS PubMed Google Scholar
Runciman, M., Darzi, A. & Mylonas, G. P. Soft robotics in minimally invasive surgery. Soft Robot. 6, 423–443 (2019).
Article PubMed PubMed Central Google Scholar
Xu, S. et al. A dynamic electrically driven soft valve for control of soft hydraulic actuators. Proc. Natl Acad. Sci. 118, e2103198118 (2021).
Article CAS PubMed PubMed Central Google Scholar
Ge, L., Dong, L., Wang, D., Ge, Q. & Gu, G. A digital light processing 3D printer for fast and high-precision fabrication of soft pneumatic actuators. Sens. Actuators A: Phys. 273, 285–292 (2018).
Li, H. et al. High-force soft pneumatic actuators based on novel casting method for robotic applications. Sens. Actuators A: Phys. 306, 111957 (2020).
Article ADS CAS Google Scholar
Bira, N., Mengüç, Y. & Davidson, J. R. In 2020 IEEE International Conference on Robotics and Automation (ICRA) (IEEE, 2020).
Acome, E. et al. Hydraulically amplified self-healing electrostatic actuators with muscle-like performance. Science 359, 61–65 (2018). This study presents muscle-mimetic soft actuators that harness electrostatic and hydraulic mechanism using liquid dielectric material.
Article ADS CAS PubMed Google Scholar
Mitchell, S. K. et al. An easy‐to‐implement toolkit to create versatile and high‐performance HASEL actuators for untethered soft robots. Adv. Sci. 6, 1900178 (2019).
Zhang, Y. F. et al. Miniature pneumatic actuators for soft robots by high‐resolution multimaterial 3D printing. Adv. Mater. Technol. 4, 1900427 (2019).
Leroy, E., Hinchet, R. & Shea, H. Multimode hydraulically amplified electrostatic actuators for wearable haptics. Adv. Mater. 32, 2002564 (2020).
Bell, M. A., Gorissen, B., Bertoldi, K., Weaver, J. C. & Wood, R. J. A modular and self‐contained fluidic engine for soft actuators. Adv. Intell. Syst. 4, 2100094 (2022).
Zatopa, A., Walker, S. & Menguc, Y. Fully soft 3D-printed electroactive fluidic valve for soft hydraulic robots. Soft Robot. 5, 258–271 (2018).
Lin, Y., Xu, Y.-X. & Juang, J.-Y. Single-actuator soft robot for in-pipe crawling. Soft Robot. 10, 174–186 (2023).
Chee, P. S., Minjal, M. N., Leow, P. L. & Ali, M. S. M. Wireless powered thermo-pneumatic micropump using frequency-controlled heater. Sens. Actuators A: Phys. 233, 1–8 (2015).
Han, J. et al. Untethered soft actuators by liquid–vapor phase transition: remote and programmable actuation. Adv. Intell. Syst. 1, 1900109 (2019).
Byun, J. et al. Underwater maneuvering of robotic sheets through buoyancy-mediated active flutter. Sci. Robot. 6, eabe0637 (2021).
Yoon, Y. et al. Bioinspired untethered soft robot with pumpless phase change soft actuators by bidirectional thermoelectrics. Chem. Eng. J. 451, 138794 (2023).
Lee, J. et al. Bioinspired soft robotic fish for wireless underwater control of gliding locomotion. Adv. Intell. Syst. 4, 2100271 (2022).
Kang, B., Lee, Y., Piao, T., Ding, Z. & Wang, W. D. Robotic soft swim bladder using liquid–vapor phase transition. Mater. Horiz. 8, 939–947 (2021).
Article CAS PubMed Google Scholar
Li, M. et al. Miniature coiled artificial muscle for wireless soft medical devices. Sci. Adv. 8, eabm5616 (2022).
Article PubMed PubMed Central Google Scholar
Mirvakili, S. M., Sim, D., Hunter, I. W. & Langer, R. Actuation of untethered pneumatic artificial muscles and soft robots using magnetically induced liquid-to-gas phase transitions. Sci. Robot. 5, eaaz4239 (2020).
Tang, Y. et al. Wireless miniature magnetic phase‐change soft actuators. Adv. Mater. 34, 2204185 (2022).
Diteesawat, R. S., Helps, T., Taghavi, M. & Rossiter, J. Electro-pneumatic pumps for soft robotics. Sci. Robot. 6, eabc3721 (2021).
Matia, Y., An, H. S., Shepherd, R. F. & Lazarus, N. Magnetohydrodynamic levitation for high-performance flexible pumps. Proc. Natl Acad. Sci. 119, e2203116119 (2022).
Article CAS PubMed PubMed Central Google Scholar
Xu, S., Nunez, C. M., Souri, M. & Wood, R. J. A compact DEA-based soft peristaltic pump for power and control of fluidic robots. Sci. Robot. 8, eadd4649 (2023).
Feng, M., Yang, D., Majidi, C. & Gu, G. High‐speed and low‐energy actuation for pneumatic soft robots with internal exhaust air recirculation. Adv. Intell. Syst. 5, 2200257 (2023).
Tse, Y. A., Wong, K. W., Yang, Y. & Wang, M. Y. In 2020 IEEE/RSJ International Conference on Intelligent Robots and Systems (IROS) (IEEE, 2020).
Sun, J., Zhou, D., Deng, J. & Liu, Y. Development of a high flow rate soft pump driven by intersected twisted artificial muscles units. IEEE Trans. Ind. Electron. 70, 7153–7162 (2022).
Zhang, W. H., Qin, L., Wang, J. Y. & Xu, W. Design of squeezing-tube-driven pump for soft pneumatic robotics based on spiral spring winding. Appl. Phys. Letters 122, 093702 (2023).
Cacucciolo, V. et al. Stretchable pumps for soft machines. Nature 572, 516–519 (2019).
Article ADS CAS PubMed Google Scholar
Tang, W. et al. Customizing a self-healing soft pump for robot. Nat. Commun. 12, 2247 (2021).
Article ADS CAS PubMed PubMed Central Google Scholar
Qi, J., Gao, F., Sun, G., Yeo, J. C. & Lim, C. T. HaptGlove—untethered pneumatic glove for multimode haptic feedback in reality–virtuality continuum. Adv. Sci. 10, 2301044 (2023).
Lin, D., Yang, F., Gong, D. & Li, R. Bio-inspired magnetic-driven folded diaphragm for biomimetic robot. Nat. Commun. 14, 163 (2023).
Article ADS CAS PubMed PubMed Central Google Scholar
Shao, Y. et al. 4D printing light-driven soft actuators based on liquid-vapor phase transition composites with inherent sensing capability. Chem. Eng. J. 454, 140271 (2023).
Fischer, P. & Ghosh, A. Magnetically actuated propulsion at low reynolds numbers: towards nanoscale control. Nanoscale 3, 557–563 (2011).
Article ADS CAS PubMed Google Scholar
Brauer, J. R. Magnetic actuators and sensors 400,1-21 (John Wiley & Sons, 2006).
Nguyen, V. Q., Ahmed, A. S. & Ramanujan, R. V. Morphing soft magnetic composites. Adv. Mater. 24, 4041–4054 (2012).
Article CAS PubMed Google Scholar
Sitti, M. & Wiersma, D. S. Pros and cons: magnetic versus optical microrobots. Adv. Mater. 32, 1906766 (2020).
Singamaneni, S., Bliznyuk, V. N., Binek, C. & Tsymbal, E. Y. Magnetic nanoparticles: recent advances in synthesis, self-assembly and applications. J. Mater. Chem. 21, 16819–16845 (2011).
Ahn, C. H. & Allen, M. G. In Proceedings IEEE Micro Electro Mechanical Systems. 1995 408 (IEEE).
Howe, D. Magnetic actuators. Sens. Actuators A: Phys. 81, 268–274 (2000).
Joyee, E. B. & Pan, Y. A fully three-dimensional printed inchworm-inspired soft robot with magnetic actuation. Soft Robot. 6, 333–345 (2019).
Kim, S., Hashi, S. & Ishiyama, K. Magnetic actuation based snake-like mechanism and locomotion driven by rotating magnetic field. IEEE Trans. Magn. 47, 3244–3247 (2011).
Peng, L. et al. Slug-inspired magnetic soft millirobot fully integrated with triboelectric nanogenerator for on‐board sensing and self‐powered charging. Nano energy 99, 107367 (2022).
Cui, J. et al. Nanomagnetic encoding of shape-morphing micromachines. Nature 575, 164–168 (2019).
Article ADS CAS PubMed Google Scholar
Gu, H. et al. Magnetic cilia carpets with programmable metachronal waves. Nat. Commun. 11, 2637 (2020).
Article ADS CAS PubMed PubMed Central Google Scholar
Al Khatib, E. et al. Magnetically actuated simple millirobots for complex navigation and modular assembly. IEEE Robot. Autom. Lett. 5, 2958–2965 (2020).
Magdanz, V. et al. IRONSperm: Sperm-templated soft magnetic microrobots. Sci. Adv. 6, eaba5855 (2020).
Article ADS CAS PubMed PubMed Central Google Scholar
Tang, D. et al. Origami-inspired magnetic-driven soft actuators with programmable designs and multiple applications. Nano Energy 89, 106424 (2021).
Mao, G. et al. Ultrafast small-scale soft electromagnetic robots. Nat. Commun. 13, 4456 (2022).
Article ADS CAS PubMed PubMed Central Google Scholar
Kim, Y. & Zhao, X. Magnetic soft materials and robots. Chem. Rev. 122, 5317–5364 (2022).
Article CAS PubMed PubMed Central Google Scholar
Wang, L. et al. Reprogrammable, magnetically controlled polymeric nanocomposite actuators. Mater. Horiz. 5, 861–867 (2018).
Cao, X. et al. 3D printing magnetic actuators for biomimetic applications. ACS Appl. Mater. Interfaces 13, 30127–30136 (2021).
Article CAS PubMed Google Scholar
Paknahad, A. A. & Tahmasebipour, M. An electromagnetic micro-actuator with PDMS-Fe3O4 nanocomposite magnetic membrane. Microelectron. Eng. 216, 111031 (2019).
Han, B. et al. Reprogrammable soft robot actuation by synergistic magnetic and light fields. Adv. Funct. Mater. 32, 2110997 (2022).
Song, H. et al. Reprogrammable ferromagnetic domains for reconfigurable soft magnetic actuators. Nano Lett. 20, 5185–5192 (2020).
Article ADS CAS PubMed Google Scholar
Mahato, M. et al. A dual‐responsive magnetoactive and electro–ionic soft actuator derived from a nickel‐based metal–organic framework. Adv. Mater. 34, 2203613 (2022).
Liu, Y. et al. Responsive magnetic nanocomposites for intelligent shape-morphing microrobots. ACS nano 17, 8899–8917 (2023).
Article CAS PubMed Google Scholar
Zhou, H., Mayorga-Martinez, C. C., Pané, S., Zhang, L. & Pumera, M. Magnetically driven micro and nanorobots. Chem. Rev. 121, 4999–5041 (2021).
Article CAS PubMed PubMed Central Google Scholar
Quashie, D. et al. Magnetic bio-hybrid micro actuators. Nanoscale 14, 4364–4379 (2022).
Gao, Y., Wei, F., Chao, Y. & Yao, L. Bioinspired soft microrobots actuated by magnetic field. Biomed. Microdevices 23, 1–19 (2021).
Abdelaziz, M. & Habib, M. in 2020 21st International Conference on Research and Education in Mechatronics (REM). 1-6 (IEEE).
Gao, W. et al. Cargo‐towing fuel‐free magnetic nanoswimmers for targeted drug delivery. small 8, 460–467 (2012).
Article CAS PubMed Google Scholar
Tang, J. et al. Super‐soft and super‐elastic DNA robot with magnetically driven navigational locomotion for cell delivery in confined space. Angew. Chem. Int. Ed. 59, 2490–2495 (2020).
Ju, Y. et al. Reconfigurable magnetic soft robots with multimodal locomotion. Nano Energy 87, 106169 (2021).
Huang, X. et al. Highly dynamic shape memory alloy actuator for fast moving soft robots. Adv. Mater. Technol. 4, 1800540 (2019).
Ishida, M. et al. Morphing structure for changing hydrodynamic characteristics of a soft underwater walking robot. IEEE Robot. Autom. Lett. 4, 4163–4169 (2019).
Xie, H. et al. Reconfigurable magnetic microrobot swarm: multimode transformation, locomotion, and manipulation. Sci. Robot. 4, eaav8006 (2019).
Ha, M. et al. Reconfigurable magnetic origami actuators with on‐board sensing for guided assembly. Adv. Mater. 33, 2008751 (2021).
Wu, Y. et al. Locally controllable magnetic soft actuators with reprogrammable contraction-derived motions. Sci. Adv. 8, eabo6021 (2022).
Article ADS CAS PubMed PubMed Central Google Scholar
Zhang, Y. et al. Reconfigurable magnetic liquid metal robot for high-performance droplet manipulation. Nano Lett. 22, 2923–2933 (2022).
Article ADS CAS PubMed Google Scholar
Guitron, S., Guha, A., Li, S. & Rus, D. 2017 IEEE International Conference on Robotics and Automation (ICRA) 4807–4813 (IEEE, 2017).
Behrens, M. R. & Ruder, W. C. Smart magnetic microrobots learn to swim with deep reinforcement learning. Adv. Intell. Syst. 4, 2200023 (2022).
Article PubMed PubMed Central Google Scholar
Cheng, Y. et al. A fast autonomous healing magnetic elastomer for instantly recoverable, modularly programmable, and thermorecyclable soft robots. Adv. Funct. Mater. 31, 2101825 (2021).
Lu, H., Hong, Y., Yang, Y., Yang, Z. & Shen, Y. Battery‐less soft millirobot that can move, sense, and communicate remotely by coupling the magnetic and piezoelectric effects. Adv. Sci. 7, 2000069 (2020).
Dong, Y. et al. Untethered small-scale magnetic soft robot with programmable magnetization and integrated multifunctional modules. Sci. Adv. 8, eabn8932 (2022).
Article CAS PubMed PubMed Central Google Scholar
Ebrahimi, N. et al. Magnetic actuation methods in bio/soft robotics. Adv. Funct. Mater. 31, 2005137 (2021).
Miriyev, A., Stack, K. & Lipson, H. Soft material for soft actuators. Nat. Commun. 8, 596 (2017).
Article ADS PubMed PubMed Central Google Scholar
Ula, S. W. et al. Liquid crystal elastomers: an introduction and review of emerging technologies. Liq. Cryst. Rev. 6, 78–107 (2018).
Küpfer, J. & Finkelmann, H. Nematic liquid single crystal elastomers. Die Makromol. Chem., Rapid Commun. 12, 717–726 (1991).
Fu, C., Xia, Z., Hurren, C., Nilghaz, A. & Wang, X. Textiles in soft robots: current progress and future trends. Biosens. Bioelectron. 196, 113690 (2022).
Article CAS PubMed Google Scholar
Xing, H., Li, J., Shi, Y., Guo, J. & Wei, J. Thermally driven photonic actuator based on silica opal photonic crystal with liquid crystal elastomer. ACS Appl. Mater. interfaces 8, 9440–9445 (2016).
Article CAS PubMed Google Scholar
Zhai, F. et al. 4D-printed untethered self-propelling soft robot with tactile perception: rolling, racing, and exploring. Matter 4, 3313–3326 (2021).
Wu, S., Hong, Y., Zhao, Y., Yin, J. & Zhu, Y. Caterpillar-inspired soft crawling robot with distributed programmable thermal actuation. Sci. Adv. 9, eadf8014 (2023).
Article CAS PubMed PubMed Central Google Scholar
Kotikian, A. et al. Untethered soft robotic matter with passive control of shape morphing and propulsion. Sci. Robot. 4, eaax7044 (2019).
He, Q. et al. Electrically controlled liquid crystal elastomer–based soft tubular actuator with multimodal actuation. Sci. Adv. 5, eaax5746 (2019).
Article ADS CAS PubMed PubMed Central Google Scholar
Jiang, H., Li, C. & Huang, X. Actuators based on liquid crystalline elastomer materials. Nanoscale 5, 5225–5240 (2013).
Article ADS CAS PubMed PubMed Central Google Scholar
Kim, T. H. et al. Biomimetic thermal-sensitive multi-transform actuator. Sci. Rep. 9, 7905 (2019).
Article ADS PubMed PubMed Central Google Scholar
Wang, H.-X. et al. Thermal-responsive hydrogel actuators with photo-programmable shapes and actuating trajectories. ACS Appl. Mater. Interfaces 14, 51244–51252 (2022).
Article CAS PubMed Google Scholar
Wei, W. et al. Recent advances and perspectives of shape memory polymer fibers. Er Poly. J. 175, 111385 (2022).
Lendlein, A. & Gould, O. E. Reprogrammable recovery and actuation behaviour of shape-memory polymers. Nat. Rev. Mater. 4, 116–133 (2019).
Yang, Y. et al. Enabling the sunlight driven response of thermally induced shape memory polymers by rewritable CH 3 NH 3 PbI 3 perovskite coating. J. Mater. Chem. A 5, 7285–7290 (2017).
Chen, S., Zhang, Q. & Feng, J. 3D printing of tunable shape memory polymer blends. J. Mater. Chem. C. 5, 8361–8365 (2017).
Qian, S., Yao, S., Wang, Y., Yuan, L. & Yu, J. Harvesting low-grade heat by coupling regenerative shape-memory actuator and piezoelectric generator. Appl. Energy 322, 119462 (2022).
Knick, C. R., Smith, G. L., Morris, C. J. & Bruck, H. A. Rapid and low power laser actuation of sputter-deposited NiTi shape memory alloy (SMA) MEMS thermal bimorph actuators. Sens. Actuators A: Phys. 291, 48–57 (2019).
Sun, J., Tighe, B., Liu, Y. & Zhao, J. Twisted-and-coiled actuators with free strokes enable soft robots with programmable motions. Soft Robot. 8, 213–225 (2021).
He, Q., Wang, Z., Wang, Y., Song, Z. & Cai, S. Recyclable and self-repairable fluid-driven liquid crystal elastomer actuator. ACS Appl. Mater. interfaces 12, 35464–35474 (2020).
Article CAS PubMed Google Scholar
Pan, M. et al. Soft actuators and robotic devices for rehabilitation and assistance. Adv. Intell. Syst. 4, 2100140 (2022).
Kanik, M. et al. Strain-programmable fiber-based artificial muscle. Science 365, 145–150 (2019).
Article ADS CAS PubMed PubMed Central Google Scholar
Shen, Q. et al. A multiple-shape memory polymer-metal composite actuator capable of programmable control, creating complex 3D motion of bending, twisting, and oscillation. Sci. Rep. 6, 24462 (2016).
Article ADS CAS PubMed PubMed Central Google Scholar
Wei, S. & Ghosh, T. K. Bioinspired structures for soft actuators. Adv. Mater. Technol. 7, 2101521 (2022).
Yang, Y., Wu, Y., Li, C., Yang, X. & Chen, W. Flexible actuators for soft robotics. Adv. Intell. Syst. 2, 1900077 (2020).
Yamada, M. et al. Photomobile polymer materials: towards light‐driven plastic motors. Angew. Chem. 120, 5064–5066 (2008).
Kumar, K. et al. A chaotic self-oscillating sunlight-driven polymer actuator. Nat. Commun. 7, 11975 (2016).
Article ADS CAS PubMed PubMed Central Google Scholar
Yang, M. et al. Bioinspired phototropic MXene‐reinforced soft tubular actuators for omnidirectional light‐tracking and adaptive photovoltaics. Adv. Funct. Mater. 32, 2201884 (2022).
Hu, Z., Zhang, Y., Jiang, H. & Lv, J.-A. Bioinspired helical-artificial fibrous muscle structured tubular soft actuators. Sci. Adv. 9, eadh3350 (2023).
Article CAS PubMed PubMed Central Google Scholar
Wani, O. M., Zeng, H. & Priimagi, A. A light-driven artificial flytrap. Nat. Commun. 8, 15546 (2017).
Article ADS CAS PubMed PubMed Central Google Scholar
Chen, Y. et al. Light-driven dandelion-inspired microfliers. Nat. Commun. 14, 3036 (2023).
Article ADS CAS PubMed PubMed Central Google Scholar
He, Q. et al. Electrospun liquid crystal elastomer microfiber actuator. Sci. Robotics 6, eabi9704 (2021).
Wang, J., Zhao, T., Fan, Y., Wu, H. & Lv, J. A. Leveraging bioinspired structural constraints for tunable and programmable snapping dynamics in high‐speed soft actuators. Adv. Funct. Mater. 33, 2209798 (2023).
Kim, I. H. et al. Human-muscle-inspired single fibre actuator with reversible percolation. Nat. Nanotechnol. 17, 1198–1205 (2022).
Article ADS MathSciNet CAS PubMed PubMed Central Google Scholar
Ionov, L. Biomimetic hydrogel‐based actuating systems. Adv. Funct. Mater. 23, 4555–4570 (2013).
Dawson, C., Vincent, J. F. & Rocca, A.-M. How pine cones open. Nature 390, 668–668 (1997).
Article ADS CAS Google Scholar
Fratzl, P. & Barth, F. G. Biomaterial systems for mechanosensing and actuation. Nature 462, 442–448 (2009).
Article ADS CAS PubMed Google Scholar
Le Duigou, A., Chabaud, G., Scarpa, F. & Castro, M. Bioinspired electro‐thermo‐hygro reversible shape‐changing materials by 4D printing. Adv. Funct. Mater. 29, 1903280 (2019).
Shin, B. et al. Hygrobot: A self-locomotive ratcheted actuator powered by environmental humidity. Sci. Robot. 3, eaar2629 (2018).
Zhang, F. et al. Unperceivable motion mimicking hygroscopic geometric reshaping of pine cones. Nat. Mater. 21, 1357–1365 (2022).
Article ADS CAS PubMed Google Scholar
Aziz, S. et al. Plant‐like tropisms in artificial muscles. Adv. Mater. 35, e2212046 (2023).
Zhao, Z. et al. Actuation and locomotion driven by moisture in paper made with natural pollen. Proc. Natl Acad. Sci. 117, 8711–8718 (2020).
Article ADS CAS PubMed PubMed Central Google Scholar
Yang, Z., An, Y., He, Y., Lian, X. & Wang, Y. A programmable actuator as synthetic earthworm. Adv. Mater. 35, 2303805 (2023).
Li, J., Mou, L., Liu, Z., Zhou, X. & Chen, Y. Oscillating light engine realized by photothermal solvent evaporation. Nat. Commun. 13, 5621 (2022).
Article ADS CAS PubMed PubMed Central Google Scholar
Li, X. et al. Bioinspired multi‐stimuli responsive actuators with synergistic color‐and morphing‐change abilities. Adv. Sci. 8, 2101295 (2021).
Wu, Y., Dong, X., Kim, J. K., Wang, C. & Sitti, M. Wireless soft millirobots for climbing three-dimensional surfaces in confined spaces. Sci. Adv. 8, eabn3431 (2022).
Article CAS PubMed PubMed Central Google Scholar
Jeong, H., Lee, J., Kim, S., Moon, H. & Hong, S. Site-specific fabrication of a melanin-like pigment through spatially confined progressive assembly on an initiator-loaded template. Nat. Commun. 14, 3432 (2023).
Article ADS CAS PubMed PubMed Central Google Scholar
Tang, W. et al. Self-contained soft electrofluidic actuators. Sci. Adv. 7, eabf8080 (2021).
Article ADS CAS PubMed PubMed Central Google Scholar
Ha, J. H. et al. Electro-responsive hydrogel-based microfluidic actuator platform for photothermal therapy. Lab a Chip 20, 3354–3364 (2020).
Ji, X. et al. An autonomous untethered fast soft robotic insect driven by low-voltage dielectric elastomer actuators. Sci. Robot. 4, eaaz6451 (2019).
Rumley, E. H. et al. Biodegradable electrohydraulic actuators for sustainable soft robots. Sci. Adv. 9, eadf5551 (2023).
Article CAS PubMed PubMed Central Google Scholar
Yan, W. et al. Origami-based integration of robots that sense, decide, and respond. Nat. Commun. 14, 1553 (2023).
Article CAS PubMed PubMed Central Google Scholar
Chi, Y., Hong, Y., Zhao, Y., Li, Y. & Yin, J. Snapping for high-speed and high-efficient butterfly stroke–like soft swimmer. Sci. Adv. 8, eadd3788 (2022).
Article PubMed PubMed Central Google Scholar
Li, T. et al. Fast-moving soft electronic fish. Sci. Adv. 3, e1602045 (2017).
Article ADS PubMed PubMed Central Google Scholar
Ankit et al. Soft actuator materials for electrically driven haptic interfaces. Adv. Intell. Syst. 4, 2100061 (2022).

Electric Actuator Ni, D. et al. Polymer interdigitated pillar electrostatic (PIPE) actuators. Microsyst. nanoengineering 8, 18 (2022).