Thank you for visiting nature.com. You are using a browser version with limited support for CSS. To obtain the best experience, we recommend you use a more up to date browser (or turn off compatibility mode in Internet Explorer). In the meantime, to ensure continued support, we are displaying the site without styles and JavaScript.
Nature Communications volume 14, Article number: 3279 (2023 ) Cite this article N,N,N',N'-Tetramethyl-1,3-propanediamine Cas no. 110-95-2

The selective transformation of a less reactive carbonyl moiety in the presence of more reactive ones can realize straightforward and environmentally benign chemical processes. However, such a transformation is highly challenging because the reactivity of carbonyl compounds, one of the most important functionalities in organic chemistry, depends on the substituents on the carbon atom. Herein, we report an Ir catalyst for the selective hydrogenolysis of urea derivatives, which are the least reactive carbonyl compounds, affording formamides and amines. Although formamide, as well as ester, amide, and carbamate substituents, are considered to be more reactive than urea, the proposed Ir catalyst tolerated these carbonyl groups and reacted with urea in a highly chemoselective manner. The proposed chemo- and regioselective hydrogenolysis allows the development of a strategy for the chemical recycling of polyurea resins.
Alice Long, Christian J. Oswood, … David W. C. MacMillan
Xiao Xie, Patrick J. Moon, … Christopher J. Chang
Shaopeng Liu, Yong Yang, … Xihe Bi
Synthetic organic chemistry creates complex molecules by repeatedly selecting and converting one of the numerous chemical bonds in a molecule1. Thus, the selective transformation of an intended functional group in an organic molecule carrying multiple functionalities is a fundamental and indispensable subject in organic synthesis. When the functional group to be reacted has a higher reactivity than any of the other functional groups present in the reactant, selective transformation can be achieved under appropriate reaction conditions and by using appropriate reagents. In other words, the selective transformation of the less reactive functional group against the generally accepted reactivity orders remains an inherent issue in state-of-the-art organic synthesis2,3,4.
Carbonyl groups are an important class of functional groups in organic chemistry and accept various nucleophiles at the carbonyl carbon to interconvert into different carbonyl compounds via nucleophilic substitution or to afford alcohols via nucleophilic addition5. Because the reactivity of the carbonyl carbon is controlled by the two substituents on the carbon atom, the relative reactivity of carbonyl compounds is strictly defined by the innate nature of the substituents (Fig. 1a)6. To change the reactivity order, some approaches using pre-7 or in situ protection8 of reactive carbonyl groups have been established (Fig. 1b). For example, the conversion of an aldehyde to an acetal (I) is often conducted to protect carbonyl groups from nucleophiles7. Alternatively, in situ protection through the tentative addition of a nucleophile to convert a carbonyl carbon to an sp3-hybridized carbon has been well studied8. In their pioneering work on in situ protection, Luche demonstrated that the combined use of NaBH4 and one equivalent of CeCl3 enables the selective reduction of ketones in the presence of aldehydes, which originates from the selective conversion of aldehydes to Ce-stabilized gem-diols (II) to resist the NaBH4 nucleophile9. Steric protection is another method for in situ protection. Yamamoto showed that capping a sterically less bulky aldehyde moiety with a sterically demanding aluminum Lewis acid (III) enables the selective addition of organolithium reagents to ketones10.
a Reactivity order of carbonyl compounds27. b Indirect synthetic approaches by protection-deprotection strategy. c Amide-selective catalytic reduction using hydrosilanes or dihydrogen. d Catalytic hydrogenolysis of urea functionality into methanol and two molecules of amines. e Chemoselective hydrogenolysis of urea derivatives into formamides and amines and unprecedented reactivity order of carbonyl compounds (This work).
While the selection of ketones over aldehydes is realized by the protection of aldehydes, the discrimination of amides over ketones/esters relies on the high basicity of the amide oxygen11,12,13,14,15. Schwartz’s reagent (Cp2Zr(H)Cl) mediates the selective reduction of amides to aldehydes, even in the presence of more reactive but less basic esters12. The activation of amides by Tf2O and subsequent reduction by Hantzsch esters afforded amines bearing ketone and ester functionalities13. When oxygen atoms can be trapped by silicon or boron atoms, selective catalytic reduction of amides over aldehydes/ketones/esters16,17,18,19,20 or ketones over aldehydes21 can be accomplished by taking advantage of the strong Si–O or B–O bonds (Fig. 1c). For example, Tinnis and Adolfsson demonstrated the reduction of amides to aldehydes using a Mo catalyst and tetramethyldisiloxane16. In this reaction, silylated aminal (IV) is proposed as an intermediate; thus, reduction can selectively afford the aldehyde as the product. The selective reduction of amides to amines has also been achieved using metal catalysts and hydrosilanes as reducing reagents16,17,18,19. In these reactions, silanes act as not only reducing reagents but also Lewis acids that selectively activate more basic amide bonds. Similarly, by adding BF3 as a Lewis acid, Ru-triphos reportedly catalyzed the selective hydrogenation of amides over esters via a boron adduct (V)20. Although catalyst-controlled changes in chemoselectivity without external Lewis acidic additives provide more straightforward synthetic strategies22, as exemplified by the chemoselectivity control of nitrogen and oxygen nucleophiles2,3,4,23,24,25,26, it is still highly challenging, especially for carbonyl compounds.
Among carbonyl compounds, urea is the least reactive27 because of stabilization by resonance from the lone pair on the nitrogen atoms to the carbonyl carbon and strong intermolecular hydrogen bonding (Fig. 1a). Therefore, the hydrogenolysis of urea in the presence of other carbonyl functionalities is one of the most challenging and unsolved chemoselectivities28. In fact, Milstein reported that a competitive reaction of urea and formamide using a Ru catalyst resulted in the selective hydrogenolysis of formamide29. Since Milstein reported the Ru-catalyzed hydrogenolysis of urea derivatives into two molecules of amine and methanol in 201129, several catalytic systems, including Ru30,31,32,33,34,35, Mn35,36,37, and Ir38, have been used for the same conversion (Fig. 1d). As formamide intermediates are more reactive than urea, urea derivatives are fully reduced to amines and methanol under these catalytic systems, with the exception using a Ru-triphos catalyst to give a mixture of formamides and amines from different diaryl- and dialkylureas31,39. In this catalytic system, however, not only urea but also ester, amide, and other carbonyl functionalities were hydrogenated under similar conditions (vide infra)31. Indeed, urea-selective hydrogenolysis in the presence of more reactive carbonyl functionalities, such as esters, has never been achieved using these catalysts and is believed to be unfeasible29,30,31,32,33,34,35,36,37,38,39.
Herein, we report the Ir-catalyzed chemoselective hydrogenolysis of urea derivatives into formamides and amines using hydrogen gas (Fig. 1e), where the urea functionality was selectively reduced even in the presence of formamide intermediates and more reactive carbonyl functionalities such as esters and amides (FG = COOEt, CONnPr2, etc.). When unsymmetric ureas were employed, the regioselective cleavage of the C–N bond was achieved. Mechanistic studies highlighted two possible reaction mechanisms for the origin of the unique chemoselectivity: (1) metal-ligand cooperative proton and hydride transfer to urea derivatives through the selective protonation of the more basic carbonyl oxygen by a proton from the ligand; and (2) thermal decomposition of urea derivatives into amines and isocyanates, the latter being selectively hydrogenated to formamide by the Ir catalyst. The selective hydrogenolysis of urea into formamide and amine serves as a strategy for the chemical recycling of polyurea resins by the transfer of molecular hydrogen.
We initiated our study using 1,3-diphenylurea (1a) as the representative substrate and explored different catalysts under a hydrogen atmosphere (2 MPa) in toluene at 130 °C (Fig. 2). With Ir complex 4 bearing the phosphine-pyrrolido 5 ligand, the hydrogenolysis of 1a occurred to afford formanilide (2a) and aniline (3a) in 82 and 83% yields, respectively. The selectivity of urea hydrogenolysis over further reduction of formanilide (2a) was calculated to be 99%, showing excellent chemoselectivity of catalyst 4. When Ir complex 6 bearing benzimidazole, which has a more acidic N–H bond than pyrrole, as the coordinating site was employed, 77% of 1a was reduced to give 2a and 3a both in 77% yields with excellent selectivity. In contrast to catalysts 4 and 6 bearing N-heterocycles, replacing the N-heterocyclic coordinating site with sulfonato (7) or carboxylato (8) groups resulted in low yields of both 2a and 3a because of the low conversion of 1a. Analogous Rh complex 9 carrying the phosphine-pyrrolido 5 ligand allowed very low conversion. Almost no reaction occurred with representative Rh and Ir hydrogenation catalysts such as Wilkinson’s catalyst 10 and Vaska’s catalyst 11. Crabtree’s catalyst 12 afforded 2a and 3a in low yields with a low selectivity of 62%. No reactions occurred without a catalyst.
Reaction conditions: 1a (0.17 mmol) and catalyst (3 mol%) in toluene (2 mL) under H2 (2 MPa) at 130 °C for 18 h. Yields were determined by 1H NMR analysis relative to an internal standard. Yield of amine 3a was reported based on the mole of 1a, being 200% at maximum. n.d.: not detected. Selectivity value = yield of 2a/{(yield of 2a + yield of 3a)/2} × 100 (%).
After optimizing the reaction conditions with 4 as the best catalyst (Supplementary Tables 1–2), we found two optimal conditions, as shown in Fig. 3a. The reaction under reduced H2 pressure (1 MPa) in THF at 130 °C (Condition A) afforded 2a and 3a in 80 and 82% yields, respectively, with excellent selectivity. Alternatively, the reaction using a catalytic amount of KOtBu as an additive in toluene (Condition B) showed higher catalytic efficiency to give 2a and 3a in 83 and 114% yields, with a slightly decreased selectivity due to the over-reduction of 2a to 3a.
All yield values were determined by 1H NMR using an internal standard. Yield of amine 3 was reported based on the mole of urea 1, being 200% at maximum. a Substrate scope of symmetric urea derivatives. *, Reaction for 48 h; †, 4 (1 mol%) and KOtBu (10 mol%) for 18 h; ‡, Reaction for 144 h; §, 4 (10 mol%) and KOtBu (33 mol%) for 48 h; ¶, 4 (3 mol%) and KOtBu (10 mol%) for 48 h. b Competitive reaction of 1a with ester 13 or carbamate 14. c Control experiments employing more reactive carbonyl compounds, ester 13 and carbamate 14. d Substrate scope of regioselective hydrogenolysis of unsymmetric urea derivatives. *, Reaction for 48 h; ¶, 4 (3 mol%) and KOtBu (10 mol%) for 48 h; #, Along with 39% yield of 3a. e Gram-scale hydrogenolysis. f Reducing catalyst loading.
With the optimized conditions in hand, the substrate scope of the hydrogenolysis was investigated (Fig. 3a; see also Supplementary Figs. 4–6). When 1,3-diarylureas bearing electron-withdrawing halogen moieties, F (1b) and Cl (1c), at the para-position were employed in the reaction, the corresponding products were obtained in high yields and selectivities. Steric hindrance had a negligible effect; meta- and ortho-substituted 1,3-diphenylureas 1d and 1e underwent hydrogenolysis to give the corresponding products, which is in sharp contrast to previous results that are largely affected by steric hindrance36. Bromo-substituted 1,3-diphenylurea 1f required 10 mol% catalyst loading for full conversion and resulted in over-reduction to give 42% yield of 2f along with 135% yield of 3f. Notably, no C–Br bond cleavage was observed under these conditions.
Electron-donating substituents such as alkyl, methoxy, and dimethylamino groups (1g–1i) slightly affected the reaction efficiency, consistent with the general electronic demand for nucleophilic addition to carbonyl compounds. Notably, the present catalyst selectively cleaved the C–N bond of the urea moiety, even for 1,3-diarylurea 1j possessing an ester moiety. This unprecedented chemoselectivity was further confirmed by the competitive reaction of 1a with ethyl benzoate (13), where selective hydrogenolysis of 1a took place, and >99% of 13 was recovered without any loss of the ester group (Fig. 3b). These results clearly show that the present catalyst possesses unique chemoselectivity that has not been reported in the literature, and that the innate reactivity order of carbonyl moieties can be reversed upon the addition of a catalytic amount of an exogenous control element. Similarly, the competitive reaction of 1a with carbamate 14 resulted in the selective hydrogenolysis of urea 1a, indicating that carbamate is less reactive than urea in the present reaction (Fig. 3b). In contrast, no selectivity was observed for the competitive reaction between urea 1a and a ketone, giving rise to a mixture of 2a, 3a, and alcohol (Supplementary Fig. 5). Therefore, the present catalyst places ureas between ketones and esters in its reactivity order, in contrast to the conventional reactivity order (Fig. 1a). The absence of observed reactions of 13 and 14 along with the over-reduction of formamides 2 in some cases suggests that the present catalyst 4 reduces formamides 2 more easily than ester 13 and carbamate 14. The amide and cyano moieties, which are believed to have higher reactivity than the urea group, were also tolerated under the reaction conditions (1k and 1l). The urea derivatives of primary and secondary alkylamines (1m and 1n) underwent hydrogenolysis with the aid of the KOtBu additive (Condition B) and afforded the products in high yields and moderate selectivities.
Control experiments using ester 13 or carbamate 14 as substrates under the optimized conditions revealed that the present catalytic system did not convert these functionalities (Fig. 3c). These results clearly differentiate our catalytic system from that using the Ru-triphos catalyst, which exhibits catalytic activity toward the hydrogenolysis of not only urea but also other carbonyl compounds such as esters, amides, and carbonates31.
Next, we focused on the hydrogenolysis of unsymmetric urea derivatives (Fig. 3d). Because two C–N bonds are cleaved in the hydrogenolysis of urea derivatives in previous reports29,30,31,32,33,34,35,36,37,38, the regioselectivity of the first C–N bond cleavage in unsymmetric ureas has not yet been addressed. When a methyl group is introduced onto the one nitrogen atom of 1,3-diphenylurea (1ao), the hydrogenolysis regioselectively occurred at the C–N bond of the secondary amine to give formanilide (2a) and N-methylaniline (3o) in 84 and 88% yields, respectively, along with small amounts of 3a (3%) under Condition A. Although exchange reaction between an amide and an amine occasionally takes place under harsh conditions7, the reaction between products 2a and 3o was negligible for urea 1ao under Condition A (Supplementary Fig. 19). When 1ao was subjected to Condition B, 2a and 3o were produced in 53 and 94% yields, respectively, along with 39% of 3a probably via the over-reduction of 2a. The introduction of an electron-withdrawing group (Cl) into the N-methylaniline moiety (1ap) or both aryl groups (1cp) did not affect the yield and regioselectivity, affording formanilides (2a or 2c) and N-methyl-4-chloroaniline (3p) in a regioselective manner. Electron-donating groups such as nBu and OMe at the para-position of either the aniline or N-methylaniline moiety (1qp and 1cr) decreased the reaction efficiency but did not affect the regio- and chemoselectivities. Lower yields were obtained following the introducing of electron-donating groups at the para-position of both aryl groups (1qr).
The reaction of unsymmetric urea 1as consisting of aniline and morpholine moieties resulted in the formation of 2a and morpholine (3s) in 12% and 14% yields, respectively, with 17% conversion of 1as under Condition A. In contrast, under Condition B, the conversion of 1as was significantly improved to >99%, and the regioselectivity was completely inverted to afford 2s and 3a in 77 and 83% yields, respectively (see the Supplementary Information, Section 1-11).
The gram-scale hydrogenolysis of 1a with 0.3 mol% 4 and 1 mol% KOtBu in THF afforded 2a and 3a in 93 and 105% yields, respectively (Fig. 3e). Further reducing the catalyst loading to 0.09 mol% resulted in the almost full conversion of 1a, which corresponds to a turn-over number of 1033 (Fig. 3f), a value more than 5-times higher than that previously reported for the hydrogenolysis of urea into amine and methanol32.
We postulated two possible reaction pathways, as shown in Fig. 4, based on mechanistic studies (for experiments and detailed discussions, see the Supplementary Information). One pathway involves metal-ligand cooperation40,41 in the hydrogenolysis process (Fig. 4a). Upon treating Ir precatalyst 4 with hydrogen gas, Ir intermediate A is formed. The heterolytic cleavage of H2 by the Ir–N bond forms complex B. The protonation of the carbonyl oxygen by the acidic N–H bond in the pyrrole moiety of B discriminates the more basic carbonyl oxygen in ureas than that in formamides (C). This is the origin of the unprecedented chemoselectivity of the present catalytic system. Subsequent hydride transfer from the Ir center or concomitant transfer of the proton and hydride to the carbonyl C=O bond forms intermediate D and regenerates Ir complex A. The elimination of one amino group from intermediate D yields an amine and a formamide in a selective manner.
a Metal-ligand cooperative pathway. b Thermal decomposition pathway. For experimental supports and detailed discussions, see the Supplementary Information.
In this catalytic cycle, the Ir center and pyrrole moiety cooperatively reduced the C=O bond of the urea moiety. Although such metal-ligand cooperation40,41 has been well established for the catalytic reduction of carbonyl compounds, including urea derivatives, the proposed catalytic cycle involves a unique mechanism, namely, the nitrogen atom in the pyrrole ring, which directly participates in the heterolytic cleavage of H2 as well as the proton transfer step.
Another possibility is the thermal decomposition of urea into isocyanate F and amine via zwitterion E prior to hydrogenation by the Ir catalyst. Isocyanate F, which is more electrophilic than formamide, is reduced by the Ir catalyst to form the formamides39,42,43 (Fig. 4b).
Control experiments and kinetic studies were consistent with these two reaction pathways, and we could not rule out one of the two possible pathways at this moment (see the Supplementary Information, Sections 1-5–1-11).
Finally, chemoselective hydrogenolysis was applied to the degradation of polyurea resins for chemical recycling44,45 (Fig. 5). Degradations of polyurea resins for chemical recycling by hydrogenolysis35,38,46,47, hydrolysis48, and solvolysis49,50 have been reported, but there are still room to improve (see the Supplementary Information, Section 1-11). Contrary, the present chemo- and regioselective catalytic hydrogenolysis enables easy separation of degraded products and realizes a novel chemical recycling strategy. The condensation reaction of diisocyanate 15 with diamine 16 afforded polyurea resin 17 with Mn = 64 × 103 as a less soluble off-white solid. The hydrogenolysis of 17 with a catalytic amount of 4 and KOtBu under slightly modified conditions mainly afforded diformamide 18 (72% yield) and diamine 16 (88% yield), the latter being one of the monomers in the formation of polyurea. The diphenylmethane unit was recovered as diformamide 18 in 72% yield, along with monoformamide 19 (24%) and diamine 20 (2%). This result is consistent with the regioselectivity observed in the hydrogenolysis of unsymmetric ureas, as exemplified by that of 1ao, and the formation of 19 and 20 could be explained by over-reduction. Notably, the obtained diformamide 18 still possesses two carbonyl groups at both ends, in contrast to the previously reported degradation of polyurea resins via hydrogenolysis35,38,46,47 that lost the carbonyl groups. Because polyurea resins can be synthesized by the dehydrogenative coupling of formamides with amines51, the combination of the present chemo- and regioselective hydrogenolysis and dehydrogenative coupling enables the chemical recycling of polyurea resins consisting of two alternating different diamine segments, as in polyurea 17, only by the transfer of molecular hydrogen (see the Supplementary Information, Section 1-12). In addition, because urea, ester, and carbamate are often found in polymer materials as polyureas, polyesters, and polyurethanes, respectively, the exceptionally high chemoselectivity of the proposed catalytic system is promising for the selective chemical recycling of polyureas from mixed polymer materials.
All yield values were determined by 1H NMR using an internal standard. PDI: poly dispersity index. See the Supplementary Information for details.
In conclusion, the Ir catalytic system allows the selective hydrogenolysis of one C–N bond in the urea functionality to afford formamides and amines as products. In addition to formamides, reactive carbonyl functionalities, such as esters, amides, and carbamates, are well tolerated under the reaction conditions. We also demonstrated the hydrogenative degradation of polyurea resins using the proposed catalytic system, in which the carbonyl carbon was retained in the degraded monomer.
The change in chemoselectivity demonstrated herein provides a new strategy for atom-economical and environmentally benign processes, unlike existing strategies that rely on stoichiometric reagents, for the selective transformation of carbonyl compounds. The combination of the proposed catalysis and dehydrogenative coupling reactions allows chemical recycling via the transfer of molecular hydrogen.
A 50 mL stainless steel autoclave, a glass tube, and a stirring bar were dried in an oven at 150 °C, and then cooled inside a glovebox under argon atmosphere. Catalyst 4 (9.4 mg, 15 µmol), urea (0.50 mmol), and THF (3 mL) were added into the glass tube, and the tube was capped with a funnel to prevent evaporation of the solvent. After the glass tube was set in the autoclave, the autoclave was sealed. The autoclave was brought out from the glovebox. The autoclave was degassed three times using H2 and was pressurized with 1 MPa of H2 for 5 min with stirring. The reaction mixture was stirred in an isothermal heating block at 130 °C for 48 h. After cooling to room temperature, H2 was vented off carefully. To the reaction mixture, DMSO-d6 (ca. 2 mL) was added to homogenize the reaction mixture, and heptane (25.1 mg, 0.25 mmol) or dibromomethane (86.9 mg, 0.50 mmol) was added as an internal standard. The solution (THF/DMSO-d6 = 3/2, 0.3 mL) was transferred into an NMR tube and diluted with DMSO-d6 (0.3 mL) for NMR analysis. The conversion of urea and the yields of formamide and amine were determined by 1H NMR spectroscopy with the internal standard (1d: heptane, others: dibromomethane). To the combined reaction mixture, ethyl acetate (30 mL) was added, and the solution was extracted with 1 M HCl aq. (20 mL × 3). The combined aqueous layers were neutralized with NaHCO3 and extracted with CH2Cl2 (50 mL × 3). The combined CH2Cl2 layers were washed with brine (100 mL), dried over anhydrous Na2SO4, and filtered. After adding 1 M HCl in MeOH, the solution was stirred at room temperature for 3 h and concentrated under reduced pressure to obtain the corresponding anilinium chloride salt. The ethyl acetate layer was washed with brine, dried over anhydrous Na2SO4, filtered, and concentrated under reduced pressure. The crude residue was purified by silica gel column chromatography with CH2Cl2/ethyl acetate as an eluent to obtain formamide.
A 50 mL stainless steel autoclave, a glass tube, and a stirring bar were dried in an oven at 150 °C, and then cooled inside a glovebox under argon atmosphere. Catalyst 4 (9.4 mg, 15 µmol), urea (0.50 mmol), KOtBu (5.6 mg, 0.05 mmol), and toluene (3 mL) were added into the glass tube, and the tube was capped with a funnel to prevent evaporation of the solvent. After the glass tube was set in the autoclave, the autoclave was sealed. The autoclave was brought out from the glovebox. The autoclave was degassed three times using H2 and was pressurized with 1 MPa of H2 for 5 min with stirring. The reaction mixture was stirred in an isothermal heating block at 130 °C for 48 h. After cooling to room temperature, H2 was vented off carefully. To the reaction mixture, DMSO-d6 (ca. 2 mL) and 0.5 M HCl aq. (0.1 mL) were added to homogenize and neutralize the reaction mixture, and dibromomethane (86.9 mg, 0.50 mmol) was added as an internal standard. The solution (toluene/DMSO-d6 = 3/2, 0.3 mL) was transferred into an NMR tube and diluted with DMSO-d6 (0.3 mL) for NMR analysis. The conversion of urea and the yields of formamide and amine were determined by 1H NMR spectroscopy with the internal standard. To the combined reaction mixture, ethyl acetate (30 mL) was added, and the solution was extracted with 1 M HCl aq. (20 mL × 3). The combined aqueous layers were neutralized with NaHCO3 and extracted with CH2Cl2 (50 mL × 3). The combined CH2Cl2 layers were washed with brine (100 mL), dried over anhydrous Na2SO4, and filtered. After adding 1 M HCl in MeOH, the solution was stirred at room temperature for 3 h and concentrated under reduced pressure to obtain the corresponding anilinium chloride salt. The ethyl acetate layer was washed with brine, dried over anhydrous Na2SO4, filtered, and concentrated under reduced pressure. The crude residue was purified by silica gel column chromatography with CH2Cl2/ethyl acetate as an eluent to obtain formamide.
A 50 mL stainless steel autoclave, a glass tube, and a stirring bar were dried in an oven at 150 °C, and then cooled inside a glovebox under argon atmosphere. Catalyst 4 (3.1 mg, 5 µmol), polyurea resin 17 (32.9 mg, 0.16 mmol/urea moiety), KOtBu (1.9 mg, 0.017 mmol), and THF (3 mL) were added into the glass tube, and the tube was capped with a funnel to prevent evaporation of the solvent. After the glass tube was set in the autoclave, the autoclave was sealed. The autoclave was brought out from the glovebox. The autoclave was degassed three times using H2 and was pressurized with 1 MPa of H2 for 5 min with stirring. The reaction mixture was stirred in an isothermal heating block at 130 °C for 18 h. After cooling to room temperature, H2 was vented off carefully. To the reaction mixture, 0.5 M HCl aq. (33 μL) was added to neutralize the reaction mixture, and dibromomethane (57.9 mg, 0.33 mmol) was added as an internal standard. The solution (0.1 mL) was transferred into an NMR tube and added with DMSO-d6 for NMR analysis. The yields of products were determined by 1H NMR spectroscopy with the internal standard (NMR yields were calculated by subtracting impurities including 17). The combined reaction mixture was evaporated and extracted with hexane (3 mL × 6). The combined hexane layers were evaporated to obtain N,N’-dimethyl-1,6-diaminohexane (16) as a colorless liquid (5.8 mg, 48%). The residue was purified by silica gel column chromatography with CH2Cl2/ethyl acetate as an eluent to obtain N,N’-(methylenebis(4,1-phenylene))diformamide (18) as a white solid (13.8 mg, 65%).
The data generated in this study are available in the main text, Supplementary Information, or Supplementary Data. Source data are available for Supplementary Figs. 16, 25, 27–31, 33–37, 39–43, and 45–49 in the associated source data file via https://doi.org/10.6084/m9.figshare.22725836. X-ray crystal data can also be obtained from the joint Cambridge Crystallographic Data Centre (CCDC: 2225764 (4), 2225765 (7), 2225766 (9), and 2225767 (23)) via https://www.ccdc.cam.ac.uk/structures/. Source data are provided with this paper.
Corey, E. J. & Cheng, X.-M. The Logic of Chemical Synthesis (Wiley, 1989).
Afagh, N. A. & Yudin, A. K. Chemoselectivity and the curious reactivity preferences of functional groups. Angew. Chem. Int. Ed. 49, 262–310 (2010).
Trader, D. J. & Carlson, E. E. Chemoselective hydroxyl group transformation: an elusive target. Mol. BioSyst. 8, 2484–2493 (2012).
Article CAS PubMed PubMed Central Google Scholar
Kristensen, T. E. Chemoselective O-acylation of hydroxyamino acids and amino alcohols under acidic reaction conditions: history, scope and applications. Beilstein J. Org. Chem. 11, 446–468 (2015).
Article CAS PubMed PubMed Central Google Scholar
Patai, S. & Zabicky, J. The Chemistry of the Carbonyl Group (Wiley, 1966).
Smith, M. B. & March, J. March’s Advanced Organic Chemistry: Reactions, Mechanisms, and Structure 6th edition (Wiley, 2007).
Wuts, P. G. M. Green’s Protective Groups in Organic Synthesis 5th edition (Wiley, 2014).
Yahata, K. & Fujioka, H. In situ protection methodology in carbonyl chemistry.Chem.Pharm.Bull.62, 1–11 (2014).
Luche, J.-L. & Gemal, A. L. Lanthanoids in organic synthesis. 5. Selective reductions of ketones in the presence of aldehydes. J. Am. Chem. Soc. 101, 5848–5849 (1979).
Maruoka, K., Saito, S., Concepcion, A. B. & Yamamoto, H. Chemoselective functionalization of more hindered aldehyde carbonyls with the methylaluminum bis(2,6-diphenylphenoxide)/alkyllithium system. J. Am. Chem. Soc. 115, 1183–1184 (1993).
Pace, V. & Holzer, W. Chemoselective activation strategies of amidic carbonyls towards nucleophilic reagents. Aust. J. Chem. 66, 507–510 (2013).
White, J. M., Tunoori, A. R. & Georg, G. I. A novel and expeditious reduction of tertiary amides to aldehydes using Cp2Zr(H)Cl. J. Am. Chem. Soc. 122, 11995–11996 (2000).
Barbe, G. & Charette, A. B. Highly chemoselective metal-free reduction of tertiary amides. J. Am. Chem. Soc. 130, 18–19 (2008).
Article CAS PubMed Google Scholar
Kornet , MJ , Thio , PA & Tan , SI The borane reduction of starch esters .J. Org.Chem.33, 3637–3639 (1968).
Dieterich, P. & Young, D. W. Synthesis of (2S,3S)-[3-2H1]- and (2S,3R)-[2,3-2H2]-proline. Tetrahedron Lett. 34, 5455–5458 (1993).
Tinnis, F., Volkov, A., Slagbrand, T. & Adolfsson, H. Chemoselective reduction of tertiary amides under thermal control: formation of either aldehydes or amines. Angew. Chem. Int. Ed. 55, 4562–4566 (2016).
Kuwano, R., Takahashi, M. & Ito, Y. Reduction of amides to amines via catalytic hydrosilylation by a rhodium complex. Tetrahedron Lett. 39, 1017–1020 (1998).
Das, S., Addis, D., Zhou, S., Junge, K. & Beller, M. Zinc-catalyzed reduction of amides: unprecedented selectivity and functional group tolerance. J. Am. Chem. Soc. 132, 1770–1771 (2010).
Article CAS PubMed Google Scholar
Cheng, C. & Brookhart, M. Iridium-catalyzed reduction of secondary amides to secondary amines and imines by diethylsilane. J. Am. Chem. Soc. 134, 11304–11307 (2012).
Article CAS PubMed Google Scholar
Yuan, M.-L., Xie, J.-H. & Zhou, Q.-L. Boron Lewis acid promoted ruthenium-catalyzed hydrogenation of amides: an efficient approach to secondary amines. ChemCatChem 8, 3036–3040 (2016).
Fujihara, T., Semba, K., Terao, J. & Tsuji, Y. Copper-catalyzed hydrosilylation with a bowl-shaped phosphane ligand: preferential reduction of a bulky ketone in the presence of an aldehyde. Angew. Chem. Int. Ed. 49, 1472–1476 (2010).
Mahatthananchai, J., Dumas, A. M. & Bode, J. W. Catalytic selective synthesis. Angew. Chem. Int. Ed. 51, 10954–10990 (2012).
Shafir, A., Lichtor, P. A. & Buchwald, S. L. N- versus O-arylation of aminoalcohols: orthogonal selectivity in copper-based catalysts. J. Am. Chem. Soc. 129, 3490–3491 (2007).
Article CAS PubMed Google Scholar
Ohshima, T., Iwasaki, T., Maegawa, Y., Yoshiyama, A. & Mashima, K. Enzyme-like chemoselective acylation of alcohols in the presence of amines catalyzed by a tetranuclear zinc cluster. J. Am. Chem. Soc. 130, 2944–2945 (2008).
Article CAS PubMed Google Scholar
Strambeanu, I. I. & White, M. C. Catalyst-controlled C–O versus C–N allylic functionalization of terminal olefins. J. Am. Chem. Soc. 135, 12032–12037 (2013).
Article CAS PubMed PubMed Central Google Scholar
Uesugi, S., Li, Z., Yazaki, R. & Ohshima, T. Chemoselective catalytic conjugate addition of alcohols over amines. Angew. Chem. Int. Ed. 53, 1611–1615 (2014).
Dub, P. A. & Ikariya, T. Catalytic reductive transformations of carboxylic and carbonic acid derivatives using molecular hydrogen. ACS Catal. 2, 1718–1741 (2012).
Cabrero-Antonino, J. R., Adam, R., Papa, V. & Beller, M. Homogeneous and heterogeneous catalytic reduction of amides and related compounds using molecular hydrogen. Nat. Commun. 11, 3893 (2020).
Article ADS PubMed PubMed Central Google Scholar
Balaraman, E., Ben-David, Y. & Milstein, D. Unprecedented catalytic hydrogenation of urea derivatives to amines and methanol. Angew. Chem. Int. Ed. 50, 11702–11705 (2011).
Miura, T., Held, I. E., Oishi, S., Naruto, M. & Saito, S. Catalytic hydrogenation of unactivated amides enabled by hydrogenation of catalyst precursor. Tetrahedron Lett. 54, 2674–2678 (2013).
vom Stein, T. et al. Highly versatile catalytic hydrogenation of carboxylic and carbonic acid derivatives using a Ru-Triphos complex: molecular control over selectivity and substrate scope. J. Am. Chem. Soc. 136, 13217–13225 (2014).
Article CAS PubMed Google Scholar
Kothandaraman, J. et al. Efficient reversible hydrogen carrier system based on amine reforming of methanol. J. Am. Chem. Soc. 139, 2549–2552 (2017).
Article CAS PubMed Google Scholar
Miura, T., Naruto, M., Toda, K., Shimomura, T. & Saito, S. Multifaceted catalytic hydrogenation of amides via diverse activation of a sterically confined bipyridine–ruthenium framework. Sci. Rep. 7, 1586 (2017).
Article ADS PubMed PubMed Central Google Scholar
Xie, Y., Hu, P., Ben-David, Y. & Milstein, D. A reversible liquid organic hydrogen carrier system based on methanol-ethylenediamine and ethylene urea. Angew. Chem. Int. Ed. 58, 5105–5109 (2019).
Liu, X. et al. Hydrogenative depolymerization of silicon-modified polyureas. Chem. Commun. 58, 5415–5418 (2022).
Das, U. K., Kumar, A., Ben-David, Y., Iron, M. A. & Milstein, D. Manganese catalyzed hydrogenation of carbamates and urea derivatives. J. Am. Chem. Soc. 141, 12962–12966 (2019).
Article CAS PubMed Google Scholar
Liu, X. & Werner, T. Indirect reduction of CO2 and recycling of polymers by manganese-catalyzed transfer hydrogenation of amides, carbamates, urea derivatives, and polyurethanes. Chem. Sci. 12, 10590–10597 (2021).
Article CAS PubMed PubMed Central Google Scholar
Kumar, A. & Luk, J. Catalytic hydrogenation of urea derivatives and polyureas. Eur. J. Org. Chem. 2021, 4546–4550 (2021).
Zhu, J. et al. Highly efficient ruthenium-catalyzed semi-hydrogenation of urea derivatives to formamides. Chem. Eur. J. e202300106, https://doi.org/10.1002/chem.202300106 (2023).
Khusnutdinova, J. R. & Milstein, D. Metal–ligand cooperation. Angew. Chem. Int. Ed. 54, 12236–12273 (2015).
Higashi, T., Kusumoto, S. & Nozaki, K. Cleavage of Si–H, B–H, and C–H bonds by metal–ligand cooperation. Chem. Rev. 119, 10393–10402 (2019).
Article CAS PubMed Google Scholar
Howell, H. G. The hydrogenation of isocyanates. Synth. Commun. 13, 635–637 (1983).
Casewit, C. J., Coons, D. E., Wright, L. L., Miller, W. K. & DuBois, M. R. Homogeneous reductions of nitrogen-containing substrates catalyzed by molybdenum(IV) complexes with µ-sulfido ligands. Organometallics 5, 951–955 (1986).
Ignatyev, I. A., Thielemans, W. & Beke, B. V. Recycling of polymers: a review. ChemSusChem 7, 1579–1593 (2014).
Article CAS PubMed Google Scholar
Coates, G. W. & Getzler, Y. D. Y. L. Chemical recycling to monomer for an ideal, circular polymer economy. Nat. Rev. Mater. 5, 501–516 (2020).
Article ADS CAS Google Scholar
Zhou, W. et al. Depolymerization of technical-grade polyamide 66 and polyurethane materials through hydrogenation. ChemSusChem. 14, 4176–4180 (2021).
Article CAS PubMed Google Scholar
Zubar, V., Haedler, A. T., Schütte, M., Hashmi, A. S. K. & Schaub, T. Hydrogenative depolymerization of polyurethanes catalyzed by a manganese pincer complex. ChemSusChem. 15, e202101606 (2022).
Article CAS PubMed Google Scholar
Motokucho, S. et al. Hydrolysis of polyurea under high pressure of carbon dioxide. Polym. Bull. 74, 615–623 (2017).
Shang, J., Liu, S., Ma, X., Lu, L. & Deng, Y. A new route of CO2 catalytic activation: syntheses of N-substituted carbamates from dialkyl carbonates and polyureas. Green Chem. 14, 2899–2906 (2012).
Li, Q., Wang, P., Liu, S., Fei, Y. & Deng, Y. Catalytic degradation of polyurea: synthesis of N-substituted carbamates with CuO–ZnO as the catalyst. Green Chem. 18, 6091–6098 (2016).
Langsted, C. R. et al. Isocyanate-free synthesis of ureas and polyureas via ruthenium catalyzed dehydrogenation of amines and formamides. J. Appl. Polym. Sci. 139, 52088 (2022).
A part of this work was conducted at Advanced Characterization Nanotechnology Platform of the University of Tokyo (JPMXP1222UT0196, −23UT0046), supported by Nanotechnology-Platform-Project by the Ministry of Education, Culture, Sports, Science & Technology (MEXT), Japan. The computations were carried out using the Research Center for Computational Science, Okazaki, Japan (Project: 22-IMS-C017, 23-IMS-C014). This work was partly supported by JST ERATO (No. JPMJER2103) to K.N., The Grant-in-Aid for Transformative Research Areas (A) JP21A204 in Digitalization-driven Transformative Organic Synthesis (Digi-TOS) from MEXT, Japan (No. JP22H05340) to T.I., The New Energy and Industrial Technology Development Organization (NEDO) (No. JPNP20004) to T.I., ENEOS TONENGENERAL foundation to T.I., and Sumitomo foundation to T.I.
These authors contributed equally: Takanori Iwasaki, Kazuki Tsuge.
Department of Chemistry and Biotechnology, Graduate School of Engineering, The University of Tokyo, 7-3-1 Hongo, Bunkyo-ku, Tokyo, 113-8656, Japan
Takanori Iwasaki, Kazuki Tsuge, Naoki Naito & Kyoko Nozaki
You can also search for this author in PubMed Google Scholar
You can also search for this author in PubMed Google Scholar
You can also search for this author in PubMed Google Scholar
You can also search for this author in PubMed Google Scholar
T.I., K.T., and N.N. performed experiments and analyzed data. T.I. and K.T. co-wrote the original draft. T.I., K.T., N.N., and K.N. reviewed and edited it.
Correspondence to Takanori Iwasaki or Kyoko Nozaki.
A Japan patent application on this work has been filed (Japan Patent Application No. 2022-020544), where K.N., T.I., K.T., and N.N. are listed as inventors.
Nature Communications thanks the anonymous reviewers for their contribution to the peer review of this work. A peer review file is available.
Publisher’s note Springer Nature remains neutral with regard to jurisdictional claims in published maps and institutional affiliations.
Open Access This article is licensed under a Creative Commons Attribution 4.0 International License, which permits use, sharing, adaptation, distribution and reproduction in any medium or format, as long as you give appropriate credit to the original author(s) and the source, provide a link to the Creative Commons license, and indicate if changes were made. The images or other third party material in this article are included in the article’s Creative Commons license, unless indicated otherwise in a credit line to the material. If material is not included in the article’s Creative Commons license and your intended use is not permitted by statutory regulation or exceeds the permitted use, you will need to obtain permission directly from the copyright holder. To view a copy of this license, visit http://creativecommons.org/licenses/by/4.0/.
Iwasaki, T., Tsuge, K., Naito, N. et al. Chemoselectivity change in catalytic hydrogenolysis enabling urea-reduction to formamide/amine over more reactive carbonyl compounds. Nat Commun 14, 3279 (2023). https://doi.org/10.1038/s41467-023-38997-2
DOI: https://doi.org/10.1038/s41467-023-38997-2
Anyone you share the following link with will be able to read this content:
Sorry, a shareable link is not currently available for this article.
Provided by the Springer Nature SharedIt content-sharing initiative
By submitting a comment you agree to abide by our Terms and Community Guidelines. If you find something abusive or that does not comply with our terms or guidelines please flag it as inappropriate.
Nature Communications (Nat Commun) ISSN 2041-1723 (online)
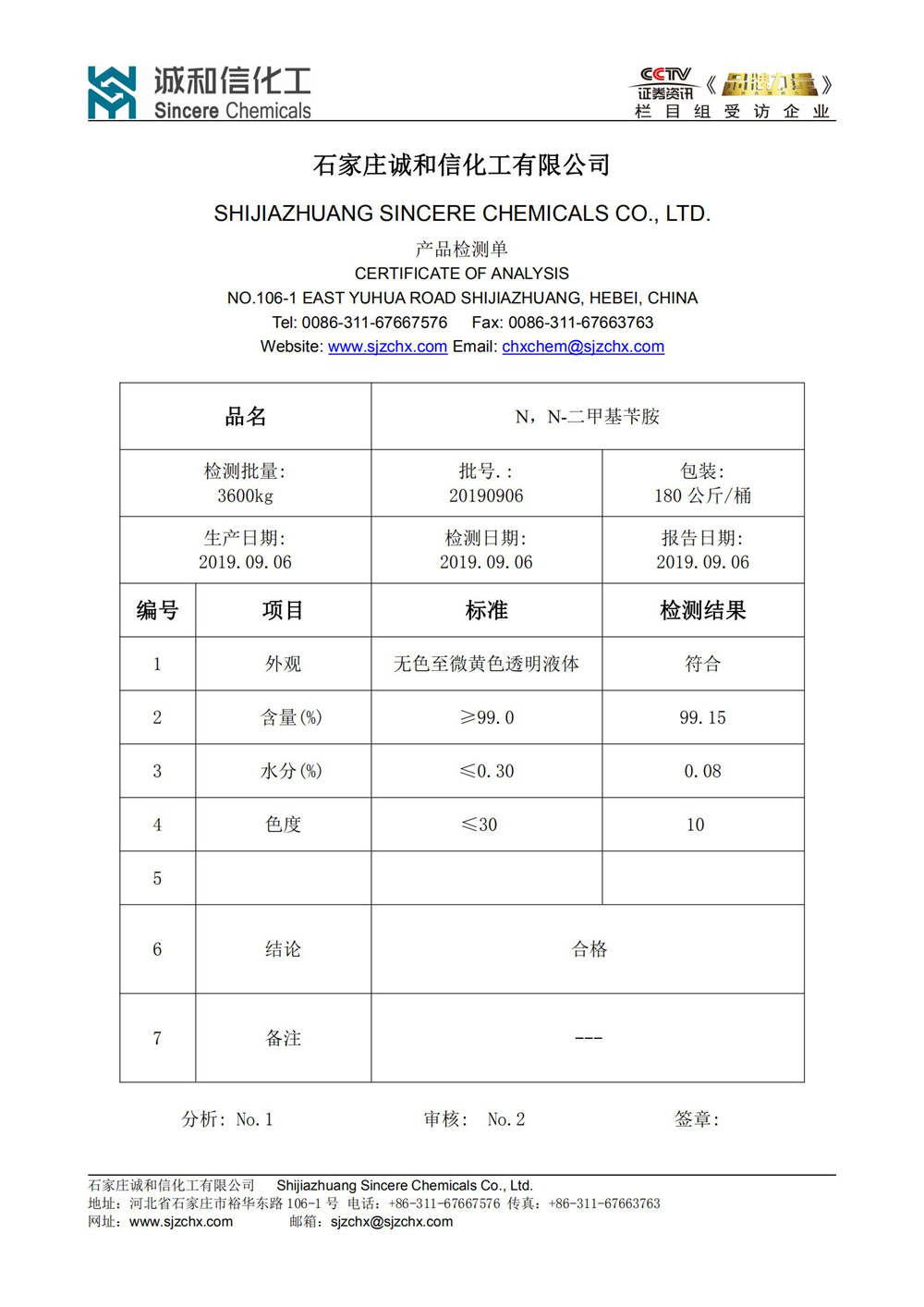
methyl piperidine CAS.No.: 35794-11-7 Sign up for the Nature Briefing newsletter — what matters in science, free to your inbox daily.