Thank you for visiting nature.com. You are using a browser version with limited support for CSS. To obtain the best experience, we recommend you use a more up to date browser (or turn off compatibility mode in Internet Explorer). In the meantime, to ensure continued support, we are displaying the site without styles and JavaScript.
Scientific Reports volume 14, Article number: 18056 (2024 ) Cite this article Acetic Acid Factory
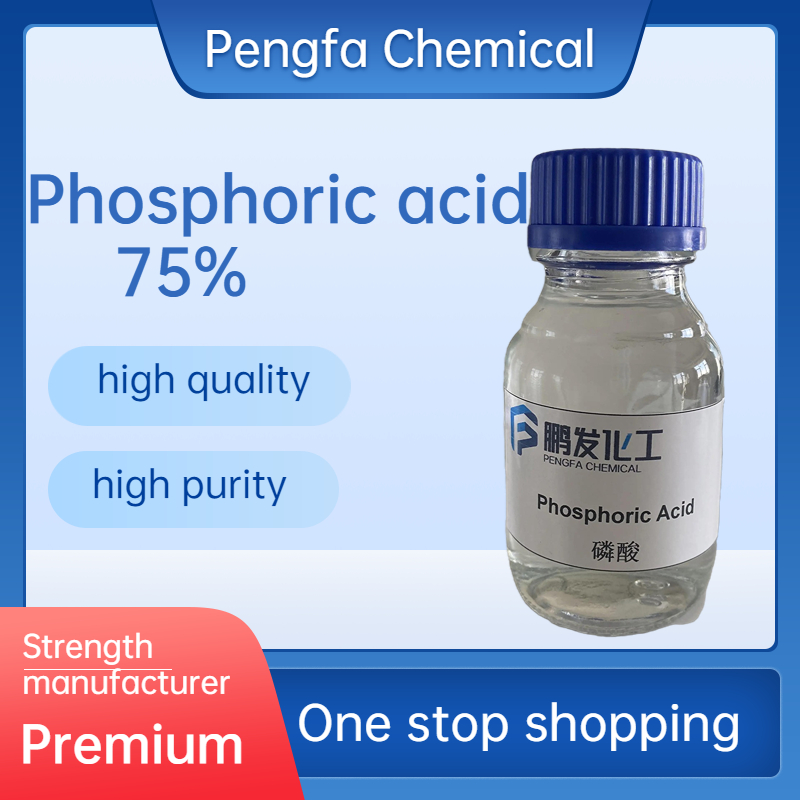
The production of date syrup yields a substantial amount of date press cake (DPC), fibrous and moisturising material with great potential for generating value through bioprocessing. However, the recalcitrant structure of DPC affects the yield of products in bioprocesses. To boost the accessibility of the structure as well as increase the soluble fraction of carbohydrates and facilitate further enzymatic hydrolysis, hydrothermal and dilute acid (0.5% (v/v) sulfuric acid) pretreatments as cost-effective and feasible methods were applied on DPC at relatively low temperatures (80, 100, 120 and 140 °C) and reaction times (60 and 90 min). The success in pretreatment was then evaluated by a post-enzymatic treatment using an enzyme cocktail of cellulases and hemicelluloses. Based on total accessible sugar with minimum produced inhibitors, an optimal operating condition was considered acid pretreatment at 120 °C for 90 min with a 55.02% increase in total sugar yield. To explore the potential use of pretreated DPC, an anaerobic digestion was conducted on untreated and acid-pretreated DPC at 120 °C for 90 min. The results showed that pretreatment increased the total bioproduct yield, including hydrogen, ethanol, and volatile fatty acid yields, by 59.75%. This demonstrates the significant impact of pretreatment on product yields in a bioprocess.
The fruit of the date palm (Phoenix dactylifera L.) is highly valued for its cultural and economic significance in the Middle East and North Africa. Date syrup, a popular product in the date processing industry, is a natural sweetener and flavouring used widely in the food industry1. The date syrup industry produces date press cake (DPC), which makes up 50% of the syrup’s weight2. Unfortunately, a significant amount of DPC is produced daily, which poses waste management difficulties since it’s either used as animal feed or disposed of in open fields and sewers. DPC is a fibrous substance with high moisture content that contains residual sugars and date pulp, which makes it easily fermentable. It’s environmentally hazardous when not handled appropriately3. DPC possesses unique characteristics that differentiate it from other lignocellulosic biomass sources. The composition of DPC can vary substantially due to different date types, processing procedures, and conditions. It contains around 0.46–6.41% protein, 0.67–5.37% fat, and 41.3–83.3% carbohydrates, including 6.67–33.81% fibres and significant amounts of cellulose, hemicellulose, and lignin3. The relatively high proportions of hemicellulose and cellulose make DPC a promising source of fermentable sugars (C5 and C6). However, the high lignin content poses challenges during enzymatic hydrolysis as it blocks enzyme access to cellulose. Using DPC as a feedstock offers environmental advantages as it utilizes a byproduct, reducing waste disposal’s ecological impact. Leveraging DPC for biomass conversion aligns with the principles of a sustainable and circular economy, providing potential environmental benefits compared to other feedstocks.
Various methods were proposed for the valorization of DPC, including the synthesis of activated carbon4,5,6; synthesis of ZnO nanoparticles7, and using as natural additive for soap production8. Studies have focused on chemical and biological processes concerning DPC, which contains a significant amount of organics that can serve as a source of carbon and nutrients for microbial bioconversion. This may result in the production of valuable products3. The citric acid2, eicosapentaenoic acid (EPA)9, and alkaline protease10 have all been produced from DPC via solid state fermentation. Submerged fermentation of DPC with different microorganisms has been used to produce glutamic acid11,12 and single-cell protein13. The production of lactic acid (LA)14,15, volatile fatty acids (VFA)14, and biohydrogen16 from DPC through dark fermentation have also been investigated.
As mentioned before, despite its potential as a biomass resource, DPC’s lignocellulosic structure limits its bioconversion potential due to its natural resistance to microbial attack17. To improve the bioconversion potential of DPC, pretreatment methods have been explored as a solution to its limitations. Pretreatment aims to increase the bio-digestibility of biomass, improve enzyme accessibility, and enhance sugar liberation during hydrolysis18. The high-temperature juice extraction process affects the lignin structure in DPC, impacting lignin removal during pretreatment and cellulose accessibility for enzymatic hydrolysis. Furthermore, pretreatment generates by-products, including acetic acid, furfural, and HMF, which can be influenced by DPC’s composition and structural features, affecting the overall efficiency of the process. Among pretreatment methods, hydrothermal and dilute sulfuric acid pretreatments are the most effective in altering lignocellulosic biomass and increasing sugar release. Still, they each have advantages and disadvantages that should be addressed19. Hemicellulose solubilization and partial lignin removal are caused by both hydrothermal and dilute sulfuric acid pretreatments, making the cellulose in biomass more accessible20. Hydrothermal pretreatment offers cost-effectiveness, no final washing or neutralization requirement, and fewer degradation products. On the other hand, dilute sulfuric acid pretreatment yields higher hemicellulose solubilization, predominantly generating monomeric sugars, making it a popular choice for industrial applications21. Hydrothermal and dilute sulfuric acid pretreatments have been examined in various investigations. Shen and Wyman22 have shown that corn stover pretreatment in 0.5% sulfuric acid at 160 °C for 40 min yielded 93.1% monomeric plus oligomeric xylose, compared to just 71.5% for hydrothermal pretreatment at 180 °C for 30 min. The study found that pretreatment at 170 °C with 2% sulfuric acid provided the highest overall process yield for pretreating olive oil pomace19. de Carvalho, et al.23 reported that sugarcane bagasse and straw form large amounts of pseudo-lignin during hydrothermal and acid pretreatments, in addition to xylan removal.
In conclusion, the unique characteristics of DPC, such as its composition, variability, solubilization behaviour, lignin structure, and inhibitor formation, set it apart from other lignocellulosic biomass sources. These distinctions directly affect pretreatment efficiency and enzyme accessibility, necessitating customized approaches to optimize biomass conversion processes. Understanding these differences and addressing the challenges posed by DPC will unlock its potential as a valuable and sustainable feedstock for producing biofuels and bioproducts.
Regarding the literature, no prior documentation of pretreatment methods in the context of DPC exists. Thus, the primary emphasis of this work was on the use of novel lignocellulosic biomass from the date extraction and syrup industry known as DPC, which is abundant and low-cost in low-temperature hydrothermal and acid pretreatment procedures to provide a suitable and economical feedstock for bioprocesses.
The primary objective of this study was to disintegrate the recalcitrant structure of DPC and facilitate the extraction of sugars in a liquid form by using feasible pretreatment techniques to create a suitable medium for bioprocessing. Hydrothermal and acid (0.5% sulfuric acid) pretreatments at low temperatures (80, 100, 120 and 140 °C) and reaction times (60 and 90 min) were applied. The effectiveness of the pretreatment method was evaluated by conducting a thorough analysis that included mass balance calculations, assessment of inhibitory compound formation, and the ease of post-enzymatic treatment using a combination of cellulase and hemicellulases. As a result, an optimal condition for pretreated DPC was identified. The pretreated DPC at optimal conditions and untreated DPC were used in anaerobic digestion to investigate the effect of pretreatment on the bioprocess.
Date press cake (DPC) was obtained from a date syrup company in Varamin, Iran. The DPC feedstock was prepared following the procedure proposed by the Biomass Analytical Procedures of the National Renewable Energy Laboratory (NREL), USA24. After collection, the samples were air-dried before being ground into a fine powder using a laboratory mill. The milled samples were sieved between 20 and 70 mesh sizes, packed in air-tight bags, and stored at 4 °C.
In the pretreatment experiment, a solid-to-liquid ratio of 1:10 (w/w) was employed, and the liquid medium, either water or 0.5% (v/v) H2SO4, was utilized. The four different temperatures were used for pretreatment were 80 °C, 100 °C, 120 °C, and 140 °C.
To maintain the temperature, the pretreatment at 80 °C was executed within a water bath using a 250 ml shake flask. For the pretreatment at 100 °C, 120 °C, and 140 °C, static conditions were adopted, and stainless-steel pressure vessels (110 ml) from Swagelok Company, Lachen, Switzerland, were employed. The temperatures were controlled using an oil bath from Julabo GmbH, Seelbach, Germany. Subsequent to the pretreatment process, the slurry was subjected to vacuum filtration, facilitating the separation of solids from liquids. The recovered fluids were preserved at − 20 °C for subsequent utilization. The recovered solids were subjected to cleaning with distilled water, drying at 70 °C, and obtaining a consistent weight.
The enzymatic hydrolysis is conducted based on the NREL method25. A 2 ml microtube (Eppendorf, Germany) was used to place the solid fraction of the pretreated DPC, which had a 10% (w/v) dry material load in 0.05 M sodium citrate buffer (pH 4.8) and a 1.5 ml working volume. Enzymatic hydrolysis was carried out using the CellicCtec2 enzyme from Novozymes A/S (Denmark) at a loading of 10 FPU/g total solids DPC. The enzyme’s activity was determined to be 154 FPU/ml according to the NREL method26. The Bicinchoninic acid assay (BCA) protein reagent kit from Pierce Biotechnology Inc., Rockford, Illinois, was used to measure the enzyme’s protein content, which was found to be 520 mg/ml. The hydrolysis tests were performed at 50 °C and 200 rpm for 24 h in a rotary shaker (Certomat-R B-Braun, Germany). All experiments were repeated three times to ensure accuracy.
In this study, batch studies for anaerobic digestion were conducted using both untreated and acid-pretreated DPC. The seeded inoculum was digested sludge with 7.76 ± 0.03% total solids (TS) and 5.43 ± 0.18% volatile solids (VS) from an anaerobic bioreactor treating wastewater (Hammar by Sjostad, Stockholm, Sweden). The inoculum was sieved and heated at 80 °C for 30 min before feeding to suppress methanogens. Glass serum vials with a 120 ml capacity and a 70 ml working volume were used for the testing. one g of substrate and 0.5 g of inoculum were used. To fill the bottles to the working volume, distilled water was utilized. The bottles were firmly closed and flushed with nitrogen gas for 2 min to provide an anaerobic environment. After that, the bottles were kept in an incubator with a water bath shaker set at 37 °C and 100 rpm for 10 days. Using a gas-tight syringe (VICI, Precision Sampling Inc., USA) to extract 250 µl of biogas during incubation, biogas composition and volume analyses were carried out.
The composition of the DPC before and after pretreatment was determined using various analytical techniques. These methods, based on the National Renewable Energy Laboratory (NREL) techniques, involved the assessment of total lignin, structural carbohydrates, total solids, and ash in the DPC27,28.
To solubilize the sample’s carbohydrates and acid-soluble lignin, acid hydrolysis with sulfuric acid was employed. Subsequently, UV spectroscopy was utilized to examine the acid-soluble lignin, while gravimetric analysis was used to calculate the amount of acid-insoluble lignin. For quantifying the monomeric sugars resulting from carbohydrate polymer hydrolysis, HPLC (Waters 2695, Waters Corporation, Milford, MA, USA) was employed.
The liquid samples were subjected to analysis for glucose, xylose, arabinose, galactose, and mannose using an HPLC system equipped with a lead (II)-based column (Aminex HPX-87P, Bio-Rad). The column was operated at 85 °C, with 0.6 ml/min Milli-Q water serving as the eluent.
To identify sugar extractives from biomass samples, the biomass was extracted with water for 2 h at a temperature of 20 °C. The liquid samples obtained after pretreatment, enzymatic saccharification and anaerobic digestion were then subjected to analysis for glucose and other sugars, and ethanol using an HPLC system (Waters Corporation, Waters 2695, Milford, Massachusetts, USA) equipped with an ion-exchange column based on hydrogen ions (Aminex HPX87-H, BioRad Laboratories, München, Germany). The column was operated at a temperature of 60 °C, with an eluent flow rate of 0.6 ml/min and 5 mM H2SO4. In anaerobic digestion samples, Using a gas-tight syringe (VICI, Precision Sampling Inc., USA), 250 μl of biogas was collected to assess its composition and volume, and in the second phase, 1 ml of the fermentation liquid was extracted using a syringe for VFAs and ethanol distribution and concentration examination.
The composition of hydrogen (H2), carbon dioxide (CO2) and methane (CH4) gas was examined using gas chromatography (GC) (Perkin-Elmer, Norwalk, CT, USA; Clarus 550). GC was used to measure the gas composition inside the serum bottles at the same pressure as the bottles themselves, and then another GC measurement was made after the gas was released from the bottles at a lower pressure inside the bottles to determine the amount of biogas produced in the bottles. The difference in peak lengths between the two observations was used to compute the amount of methane gas emitted. A packed column (CarboxenTM 1000, 6́ × 1.8́́ OD, 60/80 mesh, Supelco, Shelton, CT, USA) and a thermal conductivity detector (TCD) were included in the GC apparatus. A nitrogen gas carrier was used to run the GC–TCD at a flow rate of 30 ml/min at 75 °C, with the injection temperature set at 200 °C. Furthermore, a flame ionized detector (FID) and a capillary column (Elite-WAX ETR, 30 m × 0.32 mm × 1.00 µm, Perkin–Elmer, Shelton, CT, USA) were used in the measurement of VFAs using a GC (Clarus 550; Perkin-Elmer, Norwalk, CT, USA).
The experiments and analyses were duplicated to ensure accuracy, and MINITAB® 17 (Minitab Ltd., Coventry, UK) was utilized for statistical analysis, employing a one-way ANOVA and a 95% confidence interval. Tukey’s test was implemented for pairwise comparisons. The error bars and intervals displayed in the text, tables, and graphs were calculated as twice the standard deviation.
The recovery percentage of solids remaining after hydrothermal and acid pretreatment (expressed as wt% on dry biomass) was calculated using the weight (w) difference before and after the experiment as:
Once pretreatment was completed, the recovery percentages of cellulose, hemicellulose, and lignin were calculated from the quantities of their corresponding sugars (glucan for cellulose, xylan, galactan, and arabinan for hemicellulose, and acid-insoluble lignin and acid-soluble lignin for lignin) found in the remaining solid using Eq. (2).
The sugar yield in the liquid fraction was calculated using Eq. (3) and determined by the liquid fraction’s released sugar (glucose or xylose).
The glucan or xylan conversion after enzymatic hydrolysis (EH) was determined based on Eq. (4):
In the present study, following an evaluation of DPC composition, hydrothermal and acid pretreatment at various temperatures (80, 100, 120, and 140 °C) and reaction times (60 and 90 min) were applied to DPC. The recovery percentage and composition of residual solids and glucose, xylose, and inhibitory compounds in liquid were first investigated. The effectiveness of pretreatment employing a cellulase and hemicellulose enzyme cocktail was then assessed using enzymatic hydrolysis on the residual solid.
Table 1 summarizes the DPC composition. Hemicellulose (about 17.4%) and cellulose (about 17.0%) constitute about one-third of DPC composition, making it a potential source of C5 and C6 fermentable sugars. On the other hand, lignin, widely recognized as a critical component that raises the cost of enzymatic hydrolysis by restricting enzyme access to cellulose29, forms a significant part of DPC structure (about 53.0%). The high lignin percentage in DPC is due to the material being a byproduct of high-temperature juice extraction from dates where the lignin fraction grows as other solids are eliminated.
Regarding extractives content, the water extraction stage dissolved 11.0 ± 0.0% (4.9% glucose and 6.1% fructose). The chemical composition of DPC can vary depending on the type of dates used and the processing procedures, conditions, and efficiency applied (Oladzad et al., 2021).
The graph in Fig. 1 displays the amount of recovered solids after undergoing hydrothermal and acid pretreatment at varying temperatures and reaction times. It was observed that hydrothermal pretreatment led to greater solid recoveries compared to acid pretreatment. The solid recovery from the most severe pretreatment (140 °C and 0.5% sulfuric acid) was 33% lower than that of the least severe (at 80 °C and water as a solution).
Solid recovery after hydrothermal and acid pretreatment at different temperatures and reaction time.
The recovery of structural carbohydrates and lignin was impacted by various factors including acid utilization, reaction temperature, and time. Figure 2 presents the recovery of cellulose (glucan), hemicelluloses (xylan, galactan, and arabinan), and lignin (acid soluble, acid insoluble, and total lignin) after hydrothermal and acid pretreatments.
Structural carbohydrates and lignin recovery (%) after pretreatment: (a) hydrothermal pretreatment, 60 min; (b) hydrothermal pretreatment, 90 min; (c) acid pretreatment, 60 min; (d) acid pretreatment, 90 min.
In hydrothermal pretreatment, glucan recovery varied from 78.5% at 140 °C and 90 min (Fig. 2b) to intact at 80 °C and 60 min (Fig. 2a). In acid pretreatment, the values for glucan recovery ranged from 62.77% at 140 °C and 90 min (Fig. 2d) to 89.4% at 80 °C and 60 min (Fig. 2c). The results indicated that acid pretreatment was more efficient in altering structure and solubilization, likely due to localized hydrolysis and cellulose removal from amorphous regions30.
Hemicelluloses were the highest degraded components throughout the hydrothermal process, as seen in Fig. 2. During the hydrothermal process, hemicelluloses deteriorated first, followed by lignin decomposition at moderate temperatures, while cellulose was challenging to break down below 200 °C, according to the biomass conversion mechanism31.
Under all conditions of acid pretreatment, the breakdown of hemicellulose was visible, and its severity increased with higher temperatures. For instance, in hydrothermal pretreatment with 60 min reaction time (Fig. 2a) sugar solubilization increased by increasing the temperature from 80 to 140 °C , leading to a drop in xylan recovery from 100 to 81.1%, respectively. On the other hand, on acid pretreatment, as it is shown in Fig. 2c, by increasing the temperature from 80 to 140 °C and giving it 60 min of reaction time, the recovery of xylan significantly dropped from 83.6 to 13.3%, respectively. The decrease occurred because the heat caused the lignin protective layer around the hemicellulose fibers, to become weaker. This made it easier for sulfuric acid to break down the hemicellulose and create a substance known as xylose32. This trend was also observed for galactan and arabinan.. Increasing the reaction time impacted the final result, as seen in the decrease of xylan recovery from 13.3 to 9.3% in acid pretreatment at 140 °C for 90 min (Fig. 2d). Similar trends in sugar release during acid pretreatment were reported by Mikulski and Kłosowski33 when the exposure time was increased.
During hydrothermal and acid pretreatments, the pretreated DPC samples showed reduced lignin content compared to untreated DPC. Hydrothermal pretreatment led to a maximum decrease of total lignin in solid structure at 140 °C and 90 min by approximately 67.9% total lignin recovery. In comparison, acid pretreatment removed a maximum of 42.5% of total lignin (total lignin recovery of 57.5%), with almost 85% of acid-soluble lignin (ASL) removed at 140 °C and 90 min (Fig. 2d). ASL was more affected by pretreatment conditions, and increasing the temperature significantly affected its removal. In acid pretreatment, ASL recovery decreased from 82.1% at 80 °C and 60 min to 15.3% at 140 °C and 60 min (Fig. 2c), but it remained constant at about 15% with extended reaction time (Fig. 2d).
The recovery of acid-insoluble lignin (AIL) slightly increased with increased temperature compared to ASL recovery. However, acid pretreatment was more effective than hydrothermal pretreatment regarding AIL removal. The excellent performance of acid pretreatment is due to the primary reactions that occur in lignin. These reactions involve breaking down aryl ether linkages through acidolysis and acid-catalyzed recondensation. However, other types of links are more resistant to this process34. Similar lignin structural changes were observed during hydrothermal pretreatment by breaking lignin carbohydrate complexes (LCC) bonds and β–O–4 linkages35.
The chemistry of reactions explains why acid pretreatment is more successful than hydrothermal pretreatment in degrading DPC. Hydrothermal pretreatment provides milder acidic conditions due to organic acids generated from biomass components, leading to reactions similar to acid pretreatment but to a lesser extent34.
An investigation was conducted on the effects of hydrothermal and acid pretreatment on the chemical structure of pretreated solid residue through FTIR analysis. The analysis of the FTIR spectra provides valuable insights into the compositional changes occurring in the solid residues during acid and hydrothermal pretreatments. As an illustration, The FTIR results of hydrothermally and acid-pretreated solid residue at 140 °C and 60 min are shown in Fig. 3.
FTIR analysis of raw material (a), acid pretreated at 140 °C and 60 min (b) and hydrothermally pretreated at 140 °C and 60 min (c).
The distinctive peak at 1030 cm−1 arises from stretching C–O, C = C, and C–C–O bands in the interconnections of polysaccharides and lignin36. A decline in band intensity was observed for the pretreated sample at around 1030 cm−1, indicating lignin removal in pretreatment samples. The band observed at 1153 cm−1 indicates the presence of β-1,4-glycosidic linkages of cellulose and hemicellulose36, and its intensity is more significant in the pretreated samples, consistent with previous research37. The enhanced band intensity might be attributed to elimination hemicellulose and a portion of lignin, resulting in a higher cellulose concentration.
The lack of bands and weaker intensity at 1236 cm−1 in samples treated with acid and hydrothermal pretreatments indicates that lignin has been removed from the DPC or that acetyl groups in hemicellulose have been broken down, according to Silva et al.38.
Similarly, the bands at 1508 cm−1 reflect the vibration of the aromatic ring in lignin39, which experienced a significant decrease in intensity during hydrothermal pretreatment. The band at 1730 cm−1, corresponding to the ketone/aldehyde C = O stretch in hemicelluloses40, diminished in acid-pretreated solids, indicating the removal of hemicellulose. Additionally, the bands at 2919 cm−1 and 2850 cm−1, which provide information on lignin C–H stretching (Guo et al., 2009), exhibited considerable intensity reduction after hydrothermal and acid pretreatments.
In hydrothermal and processed solids, the intensity of the bands in 3320 cm−1 was reduced. The absorption band ranging from 3200 to 3600 cm−1 corresponds to the stretching of –OH groups found in alcohols, carboxylic acids, and hydroperoxide. This is associated with hemicellulose40. The fact that the band intensity decreased indicated that the pretreatment procedures were successful in dissolving the mentioned bands.
The chemical alterations in the structure of DPC based on differences in peak intensity were seen in both acid-pretreated and hydrothermal-pretreated solid residues. Overall, FTIR verified the NREL method’s results for solid residue composition.
The pretreatment process generates a diverse range of by-products, which vary depending on the technology and feedstock used41. The analysis focused on the liquid fractions to examine the presence of monomeric sugars and degradation products such as acetic acid, furfural, HMF (hydroxymethylfurfural), formic acid, and levulinic acid. The concentration of xylose, which is the primary component of hemicellulose, was examined in the liquid fraction along with glucose, representing the cellulose component. Figure 4a, b present the sugar concentrations and the levels of degradation by-products resulting from the DPC pretreatment process at different temperatures. The primary derivatives observed were acetic acid, furfural, and HMF.
Liquid fraction composition (a) sugar yields in liquid fraction after hydrothermal and acid pretreatment, (b) inhibitor concentration in the liquid fraction.
According to liquid fraction analysis (Fig. 4a), neither hydrothermal nor acid pretreatment significantly influenced glucan concentration in liquors (p-value = 0.282). The results of the solid fraction on glucan recovery further showed that following hydrothermal and acid pretreatment, the glucan hydrolysis to glucose was modest, and the solid fraction of pretreated material retains a large portion of the glucan present in DPC. Hence no significant changes in glucose concentration were detected.
The study showed that the liquid fraction released during pretreatment contained more xylose in acid pretreatment as the temperature and reaction time increased. This increase was due to the hemicellulose component. In all pretreatment procedures, the results are consistent with lower xylan recovery at higher temperatures and reaction times. The utilization of 0.5% sulfuric acid resulted in a rise in xylose content in all samples, indicating that hemicellulose hydrolysis increased which is consistent with solid fraction characterization results. However, there is a sugar loss when the decreased sugar from the solid structure is compared to the sugar released in the liquid portion. This might be due to a variety of factors. Firstly, after hydrothermal pretreatments, sugar exists in oligomeric form in the liquid fractions, but this form cannot be detected by HPLC34. In acidic conditions, xylose can be obtained in its form without the need to break down oligosaccharides. This is useful when monomeric xylose is needed as a source of carbon19. Secondly, certain sugars that have deteriorated in the liquid portion are transformed into substances that inhibit the process, such as acetic acid, HMF, and furfural, which can be seen that in hydrothermal pretreatement the xylose yield is lower at higher tempretures as the HMF and acetic acid formation is occured Generally, acetic acid is produced from the disintegration of acetyl groups in hemicellulose, while furfural and HMF are generated from the dehydration of pentose and hexose sugars42.
When subjected to higher hydrothermal pretreatment temperatures, a greater quantity of acetic acid was detected, which can be attributed to the breakdown of sugars42. The amount of acetic acid in liquid fractions varies substantially depending on the maximum temperature used in hydrothermal pretreatment, with significant amounts of acetic acid (0.41 g/L) formed at 140 °C compared to other temperatures. In acid pretreatment, the amount of produced acetic acid was higher compared to hydrothermal pretreatment. Acetic acid was detected under all conditions, and its concentration increased from 0.17 to 1.48 g/L as the temperature was raised from 80 to 140 °C during a 60 min reaction time. The level of acetic acid rose slightly when the reaction time was prolonged from 60 to 90 min. However, this increase was not considered statistically significant with a p-value of 0.578. Acetic acid is a common by-product resulting from the hydrolysis of acetylated xylan in hemicellulose during acid pretreatment43. Since acid pretreatment mainly affects hemicellulose, more hemicellulose is solubilized and released into the liquid fraction44. As a result, the liquid fraction produced from acid pretreatment contains more acetic acid than that from hydrothermal pretreatment. These findings align with previous studies, which have observed that acid pretreatment produces significantly more acetic acid compared to water-based pretreatment41.
HMF was detected in both hydrothermal and acid pretreatment, with its concentration increasing as the temperature was raised. After 90 min at a temperature of 120 °C, the concentration of HMF reached 0.018 g/L. Increasing the temperature to 140 °C caused the concentration of HMF to increase to 0.28 g/L. When 0.5% sulfuric acid was supplemented at a temperature of 140 °C, however, HMF content increased by around 4.3 times, from 0.28 g/L in hydrothermal pretreatment and 90 min reaction time to 1.2 g/L in acid pretreatment and 90 min reaction time. Based on the research results, it was concluded that the HMF concentration is not significantly impacted by reaction time (p-value = 0.664). HMF can be produced by degrading d -glucose derived from cellulose or hexoses derived from hemicellulose45. The production of HMF can also occur directly from fructose, which plays a crucial role in the synthesis process of HMF from glucose. Based on studies of kinetics, the transformation of fructose into HMF happens more rapidly than the conversion of glucose. Therefore, materials containing fructose are an important substrate for the synthesis of HMF46. As fructose is responsible for a portion of the sugar mix concentration in samples, it appears that it is also responsible for a part of HMF generation. Since the amount of glucose and other hexoses released from solids increases due to the acid pretreatment, the HMF concentration rises dramatically.
Furfural was only detected in liquid fractions from acid pretreatment at 140 °C. By extending the reaction time from 60 to 90 min, the concentration of furfural rose from 0.16 to 0.35 g/L The presence of furfural in the liquid portion of acid-pretreated samples is owed to a higher concentration of pentose sugars, specifically xylose. Furfural is produced by the pentoses, mainly d -xylose and l -arabinose, which are released from hemicellulose45. Furfural-producing processes are effectively carried out in an acidic condition, where the polymeric pentosan molecules may be broken up rather quickly during the hydrolysis phases. Furfural was likewise found only at the highest applied temperature of 140 °C because at higher temperatures, furfural yields and reaction rates increase as the H+ ion concentration rises47.
Regardless of the pretreatment method, no formic or levulinic acid was detected in the liquid fractions. Additionally, all samples had a production of acetic acid, furfural, and HMF that was below the level of fermentation inhibition48.
The main objective of biomass pretreatment is to decrease biomass recalcitrance, facilitating the efficient conversion of biomass into fermentable monosaccharides and other essential chemical compounds during the enzymatic hydrolysis phase49. As a result, the subsequent step involved investigating the impact of hydrothermal and acid pretreatment on the enzymatic digestibility of the solids produced after pretreatment using enzymatic saccharification assays. Figure 5a, b illustrate the outcomes of enzymatic saccharification yields for glucan and xylan, comparing them to untreated DPC.
(a) Glucan conversion (%) and (b) xylan conversion (%) after enzymatic hydrolysis of pretreated solids.
Acid pretreated solid at 140 °C and 90 min reaction time had considerably greater saccharification yields, with cellulose digestibility reaching 71.4% after 24 h. In contrast, hydrothermally pretreated DPC at the same temperature and reaction time only got 53.8% over the same period. The results showed that acid pretreatment at 140 °C improved cellulose enzymatic hydrolysis by up to 44% compared to the untreated sample. There was no statistically significant difference between glucan conversion in different reaction times of 60 and 90 min (p-value = 0.135). However, there was a meaningful difference between glucan conversion at different temperatures (p-value = 0.028). Enzyme binding to the cellulose surface is a crucial factor for enzymatic hydrolysis50. Pretreatment is also expected to enhance the accessibility and surface area (increased structural porosity) of biomass, making it more amenable to enzymatic attack. However, a significant amount of cellulose (28.6% glucose in the harshest pretreatment condition) remained unhydrolyzed and present in the solid residue. This is likely due to the high residual lignin content, although lignin’s specific mechanism of enzyme inhibition may be challenging to characterize. Nevertheless, other inhibitory mechanisms related to lignin have been suggested, such as cellulose association with lignin, hindering the enzyme’s access to cellulose, and irreversible binding of enzymes to lignin51.
The maximum xylan conversion was achieved at 140 °C and 90 min of acid pretreatment (28.4%), followed by 24.2% at 120 °C and 90 min of acid pretreatment after 24 h. However, in other conditions, xylan conversion is less than 12%. So, it appears that acid pretreatment at 140 °C is effective in hemicellulose solubilization and structural destruction.
After undergoing acidic pretreatment, lignin appears to undergo chemical rearrangement, which is believed to have a crucial impact on enzymatic hydrolysis. In more severe conditions, during the acid pretreatment, lignin deposition occurs on the residue surface, and the amount of this deposition depends on the temperature used in the pretreatment process. The exposed lignin then acts as a physical barrier, hindering the movement of enzymes and reducing glucose yield during enzymatic hydrolysis50. However, interestingly, when using sulfuric acid in the pretreatment process, the hydrolysis efficiency significantly improves with an increase in the duration of pretreatment at a given temperature.
A total mass balance accounting for the sugars entering, being converted, and leaving the pretreatment system was used to evaluate the process efficiency for glucan and xylan recovery. The total sugar yield, as shown in Fig. 6, was calculated using the release of glucose and fructose in extractives, glucose, and xylose in the pretreatment step, followed by enzymatic hydrolysis, and yield of consumed pentoses and hexoses for the production of inhibitors including HMF, furfural, and acetic acid. As shown in Fig. 6, the highest sugar yield was obtained after acid pretreatment at 140 °C. By raising the temperature during acid pretreatment, not only did the amount of total sugar increase but also did the concentration of inhibitors. Around 22% of total released sugar was used to form inhibitors after acid pretreatment at 140 °C for 90 min. However, roughly 10% of total sugar is spent for inhibitor formation at 120 °C and 90 min of acid pretreatment, and total available sugar is 8% less than total available sugars obtained at 140 °C.
During all hydrothermal pretreatment conditions, the quantity of sugar liberated by removing the total amount of sugar consumed for inhibitors is nearly consistent, as well as a small amount of sugar consumed for acetic acid formation.
Overall, acid pretreatment increases the quantity of total sugar by up to 74%, whereas hydrothermal pretreatment increases it by up to 53%. However, considering the amount of sugar consumed for inhibitor formation, the maximum increase in total available sugar for acid and hydrothermal pretreatments is around 58% and 48%, respectively. As a result, acid pretreatment was found to be more effective in sugar release.
The effect of acid pretreatment on DPC at 120 °C for 90 min was investigated to comprehend the influence of pretreatment on anaerobic digestion. In this phase, the acid-pretreated DPC—composed of both liquid and solid—was compared with the untreated DPC to examine the impact of substrate pretreatment on product yields. Using both pretreated and untreated substrates, a 10 day anaerobic digestion at pH 6 with the primary goals being the formation of VFA, gas (methane, hydrogen), and ethanol was conducted. Figure 7 shows the findings from our investigation.
Yield of products from anaerobic digestion of (a) untreated DPC, (b) acid-pretreated DPC at 120 °C and 90 min.
By analysing the produced gas, it was found that pretreatment significantly affects the amount of hydrogen produced. Raw DPC produced the greatest hydrogen yield of 6.7 ml/g VS, whereas pretreatment produced the highest yield of 107 ml/g VS. Vanyan, et al.52 found that pretreating wasted coffee grounds with diluted sulfuric acid enhanced the formation of biohydrogen during anaerobic digestion. No mthane was detected in the environment for both condition. The pH level of the environment has a significant impact on the metabolic pathways preferred by microbial communities. In acidic conditions, hydrogenogenesis is usually favored, while methane production is favored in neutral to slightly alkaline pH. The pH conditions in the anaerobic digester affect the distribution of microorganisms that produce hydrogen and methane, which in turn affects the production rates of these gases53. It should also be noticed that heat shocked inoculum was used which could inhibit the activity of acetoclastic methanogens. previous studies have demonstrated that this pretreatment method of inoculum effectively reduces methanogenic activity54,55.
The utilization of pretreated substrate has been found to result in a substantial increase in VFA yield. This enhancement is approximately 1.3 times greater than that of untreated substrate, clearly demonstrating the positive impact of pretreatment on VFA production. Numerous studies have confirmed that pretreatment of lignocellulosic substrate can significantly boost VFA production during anaerobic digestion. For instance, a study reported that hydrothermally pretreated rice straw led to a 38% increase in VFA production56, while another study found that hydrothermal pretreatment of corn stover increased VFA production by 31%57.
According to our findings, pretreating the substrate resulted in a significant increase in ethanol production. This increase was about 2.5 times more than that of the untreated substrate. Bondesson, et al.58 also found that the highest ethanol yield was obtained from the material pretreated in the presence of sulfuric acid.
Following ten days of anaerobic digestion, the yield of all bioproducts was assessed. It was found that the pretreated DPC had a considerable improvement in yield, from 0.25 g/g VS for raw DPC to 0.39 g/g VS for pretreated DPC. This means that there was a 59.75% increase in total bioproduct yield, which shows that pretreatment was effective in enhancing the enzymatic hydrolysis of the substrate. As mentioned in the previous section, there was also a 55% increase in total accessible sugar content, which further supports the effectiveness of pretreatment. These results highlight the positive impact of pretreatment in different aspects of anaerobic digestion, providing valuable insights for optimizing bioenergy production processes.
This study examined the effects of hydrothermal and acid pretreatment on DPC for bioprocessing applications. It was discovered that acid pretreatment considerably improved sugar release compared to hydrothermal pretreatment by altering the cellulose structure, solubilizing cellulose and removing the lignin.
However, it is crucial to take into account the development of inhibitors during pretreatment, since some of the released sugar was utilized to produce inhibitors. Finding a balance between inhibitor formation and sugar release is essential for optimizing pretreatment conditions for biomass conversion. The enzymatic hydrolysis results also showed that acid pretreatment at higher temperatures and longer reaction times increased cellulose’s digestibility even with residual lignin up to 71.4%. Using acid-pretreated DPC (at 120 °C and 90 min reaction time) in an anaerobic digestion process, it was observed that the yield of bioproducts rose by 59.75%, which is in line with a 58% rise in total accessible sugar release.
Once taken into account, these observations offer insightful advice for improving the DPC pretreatment procedure. The goal is to turn DPC into a viable feedstock for value-added product manufacture and bioprocesses including anaerobic digestion which can be enhanced more economically and efficiently by optimizing inhibitor production and sugar recovery.
This published paper includes all the data produced or analyzed during the study.
Djaoud, K. et al. Dairy dessert processing: Effect of sugar substitution by date syrup and powder on its quality characteristics. J. Food Process. Preserv. 44, e14414 (2020).
Mazaheri, A. M. & Nikkhah, M. Production of citric acid from date pulp by solid state fermentation. J. Agric. Sci. 4, 119–125 (2002).
Oladzad, S., Fallah, N., Mahboubi, A., Afsham, N. & Taherzadeh, M. J. Date fruit processing waste and approaches to its valorization: A review. Bioresour. Technol. 340, 125625 (2021).
Article CAS PubMed Google Scholar
Norouzi, S. et al. Preparation, characterization and Cr (VI) adsorption evaluation of NaOH-activated carbon produced from date press cake; an agro–industrial waste. Bioresour. Technol. 258, 48–56 (2018).
Article CAS PubMed Google Scholar
Heidarinejad, Z., Rahmanian, O., Fazlzadeh, M. & Heidari, M. Enhancement of methylene blue adsorption onto activated carbon prepared from date press cake by low frequency ultrasound. J. Mol. Liq. 264, 591–599 (2018).
Hasanzadeh, V., Rahmanian, O. & Heidari, M. Cefixime adsorption onto activated carbon prepared by dry thermochemical activation of date fruit residues. Microchem. J. 152, 104261 (2020).
Rambabu, K., Bharath, G., Banat, F. & Show, P. L. Green synthesis of zinc oxide nanoparticles using Phoenix dactylifera waste as bioreductant for effective dye degradation and antibacterial performance in wastewater treatment. J. Hazard. Mater. 402, 123560 (2021).
Article CAS PubMed Google Scholar
Rambabu, K. et al. Date-fruit syrup waste extract as a natural additive for soap production with enhanced antioxidant and antibacterial activity. Environ. Technol. Innov. 20, 101153 (2020).
Asadi, S. Z., Khosravi-Darani, K., Nikoopour, H. & Bakhoda, H. Production of arachidonic acid and eicosapentaenoic acid by Mortierella alpina CBS 528.72 on date waste. Food Technol. Biotechnol. 56, 197 (2018).
Article CAS PubMed PubMed Central Google Scholar
Khosravi-Darani , K. , Falahatpishe , HR & Jalali , M. Alkaline protease production on date waste by an alkalophilic Bacillus sp . 2-5 isolated from soil. Afr. J. Biotechnol. 7, 1536–1542 (2008).
Tavakkoli, M., Hamidi-Esfahani, Z. & Azizi, M. H. Optimization of Corynebacterium glutamicum glutamic acid production by response surface methodology. Food Bioprocess Technol. 5, 92–99 (2012).
Davati, N., Hamidi, E. Z. & Shojaosadati, S. A. Optimization of medium composition for microbial production of glutamic acid from Date fruit wastes using fractional factorial method. Iran. J. Food Sci. Technol. 7(2), 61–67 (2010).
Al-Farsi, M., Al Bakir, A., Al Marzouqi, H. & Thomas, R. Production of single cell protein from date waste. By Prod. Palm Trees Appl. 11, 302 (2019).
Ahmad, A., Banat, F. & Taher, H. Comparative study of lactic acid production from date pulp waste by batch and cyclic–mode dark fermentation. Waste Manag. 120, 585–593 (2021).
Article CAS PubMed Google Scholar
Ahmad, A., Othman, I., Taher, H. & Banat, F. Lactic acid recovery from date pulp waste fermentation broth by ions exchange resins. Environ. Technol. Innov. 22, 101438 (2021).
Rambabu, K. et al. Ferric oxide/date seed activated carbon nanocomposites mediated dark fermentation of date fruit wastes for enriched biohydrogen production. Int. J. Hydrog. Energy 46, 16631–16643 (2021).
Article ADS CAS Google Scholar
Wyman, C. E. et al. Comparative sugar recovery and fermentation data following pretreatment of poplar wood by leading technologies. Biotechnol. Prog. 25, 333–339 (2009).
Article CAS PubMed Google Scholar
Taherzadeh, M. J. & Karimi, K. Pretreatment of lignocellulosic wastes to improve ethanol and biogas production: A review. Int. J. Mol. Sci. 9, 1621–1651 (2008).
Article CAS PubMed PubMed Central Google Scholar
Manzanares, P. et al. Processing of extracted olive oil pomace residue by hydrothermal or dilute acid pretreatment and enzymatic hydrolysis in a biorefinery context. Renew. Energy 145, 1235–1245 (2020).
Agbor, V. B., Cicek, N., Sparling, R., Berlin, A. & Levin, D. B. Biomass pretreatment: Fundamentals toward application. Biotechnol. Adv. 29, 675–685 (2011).
Article CAS PubMed Google Scholar
Tanjore, D., Shi, J. & Wyman, C. E. Dilute acid and hydrothermal pretreatment of cellulosic biomass. In Chemical and Biochemical Catalysis for Next Generation Biofuels 64–88 (The Royal Society of Chemistry, 2011).
Shen, J. & Wyman, C. E. A novel mechanism and kinetic model to explain enhanced xylose yields from dilute sulfuric acid compared to hydrothermal pretreatment of corn stover. Bioresour. Technol. 102, 9111–9120 (2011).
Article CAS PubMed Google Scholar
de Carvalho, D. M. et al. Assessment of chemical transformations in eucalyptus, sugarcane bagasse and straw during hydrothermal, dilute acid, and alkaline pretreatments. Ind. Crops Prod. 73, 118–126 (2015).
Hames, B. et al. Preparation of samples for compositional analysis. Lab. Anal. Proced. 1617, 65–71 (2008).
Resch, M. G., Baker, J. & Decker, S. Low Solids Enzymatic Saccharification of Lignocellulosic Biomass (National Renewable Energy Laboratory, 2015).
Adney, B. & Baker, J. Measurement of Cellulase Activities. Technical Report, NREL/TP-510-42628 (National Renewable Energy Laboratory, Golden, CO, 2008).
Sluiter, A. et al. Determination of structural carbohydrates and lignin in biomass. Lab. Anal. Proced. 1617, 1–16 (2008).
Sluiter, A. et al. Biomass and total dissolved solids in liquid process samples. In Laboratory Analytical Procedure (LAP) (National Renewable Energy Laboratory (NREL), 2008).
Sheng, Y. et al. Enzymatic conversion of pretreated lignocellulosic biomass: A review on influence of structural changes of lignin. Bioresour. Technol. 324, 124631 (2021).
Article CAS PubMed Google Scholar
Foston, M. & Ragauskas, A. J. Changes in lignocellulosic supramolecular and ultrastructure during dilute acid pretreatment of populus and switchgrass. Biomass Bioenergy 34, 1885–1895 (2010).
Ando, H. et al. Decomposition behavior of plant biomass in hot-compressed water. Ind. Eng. Chem. Res. 39, 3688–3693 (2000).
Liu, X., Lu, M., Ai, N., Yu, F. & Ji, J. Kinetic model analysis of dilute sulfuric acid-catalyzed hemicellulose hydrolysis in sweet sorghum bagasse for xylose production. Ind. Crops Prod. 38, 81–86 (2012).
Mikulski, D. & Kłosowski, G. Efficiency of dilute sulfuric acid pretreatment of distillery stillage in the production of cellulosic ethanol. Bioresour. Technol. 268, 424–433 (2018).
Article CAS PubMed Google Scholar
Pu, Y., Hu, F., Huang, F., Davison, B. H. & Ragauskas, A. J. Assessing the molecular structure basis for biomass recalcitrance during dilute acid and hydrothermal pretreatments. Biotechnol. Biofuels 6, 1–13 (2013).
Sun, Q. et al. Ultrastructural change in lignocellulosic biomass during hydrothermal pretreatment. Bioresour. Technol. 341, 125807 (2021).
Article CAS PubMed Google Scholar
Wilson, R. H. et al. The mechanical properties and molecular dynamics of plant cell wall polysaccharides studied by Fourier-transform infrared spectroscopy. Plant Physiol. 124, 397–406 (2000).
Article CAS PubMed PubMed Central Google Scholar
Sahoo, D. et al. Effect of dilute acid pretreatment of wild rice grass (Zizania latifolia) from Loktak Lake for enzymatic hydrolysis. Bioresour. Technol. 253, 252–255 (2018).
Article CAS PubMed Google Scholar
Silva, T. A. L. et al. Effect of steam explosion pretreatment catalysed by organic acid and alkali on chemical and structural properties and enzymatic hydrolysis of sugarcane bagasse. Waste Biomass Valoriz. 9, 2191–2201 (2018).
Subhedar, P. B. & Gogate, P. R. Alkaline and ultrasound assisted alkaline pretreatment for intensification of delignification process from sustainable raw-material. Ultrason. Sonochem. 21, 216–225 (2014).
Article CAS PubMed Google Scholar
Ravindran, R., Jaiswal, S., Abu-Ghannam, N. & Jaiswal, A. K. Two-step sequential pretreatment for the enhanced enzymatic hydrolysis of coffee spent waste. Bioresour. Technol. 239, 276–284 (2017).
Article CAS PubMed Google Scholar
Ladeira-Ázar, R. I. S., Morgan, T., Maitan-Alfenas, G. P. & Guimarães, V. M. Inhibitors compounds on sugarcane bagasse saccharification: Effects of pretreatment methods and alternatives to decrease inhibition. Appl. Biochem. Biotechnol. 188, 29–42 (2019).
Zakaria, M. R., Hirata, S. & Hassan, M. A. Hydrothermal pretreatment enhanced enzymatic hydrolysis and glucose production from oil palm biomass. Bioresour. Technol. 176, 142–148 (2015).
Article CAS PubMed Google Scholar
Liu, X. et al. Quantification of glucose, xylose, arabinose, furfural, and HMF in corncob hydrolysate by HPLC-PDA–ELSD. Carbohydr. Res. 353, 111–114 (2012).
Article ADS CAS PubMed Google Scholar
Solarte-Toro, J. C. et al. Acid pretreatment of lignocellulosic biomass for energy vectors production: A review focused on operational conditions and techno–economic assessment for bioethanol production. Renew. Sustain. Energy Rev. 107, 587–601 (2019).
Rasmussen, H., Sørensen, H. R. & Meyer, A. S. Formation of degradation compounds from lignocellulosic biomass in the biorefinery: Sugar reaction mechanisms. Carbohydr. Res. 385, 45–57 (2014).
Article CAS PubMed Google Scholar
Jung, D., Körner, P. & Kruse, A. Kinetic study on the impact of acidity and acid concentration on the formation of 5-hydroxymethylfurfural (HMF), humins, and levulinic acid in the hydrothermal conversion of fructose. Biomass Convers. Biorefin. 11, 1155–1170 (2021).
Bamufleh, H. S., Alhamed, Y. A. & Daous, M. A. Furfural from midribs of date-palm trees by sulfuric acid hydrolysis. Ind. Crops Prod. 42, 421–428 (2013).
Gong, C. S., Cao, N. J., Du, J. & Tsao, G. T. Ethanol production from renewable resources. Recent Prog. Bioconvers. lignocellul. https://doi.org/10.1007/3-540-49194-5_9 (1999).
Chandra, R. P. et al. Substrate pretreatment: The key to effective enzymatic hydrolysis of lignocellulosics?. Biofuels https://doi.org/10.1007/10_2007_064 (2007).
Yang, H., Wang, K., Xu, F., Sun, R.-C. & Lu, Y. H2SO4-catalyzed hydrothermal pretreatment of triploid poplar to enhance enzymatic hydrolysis. Ind. Eng. Chem. Res. 51, 11598–11604 (2012).
Lee, I. & Yu, J.-H. The production of fermentable sugar and bioethanol from acacia wood by optimizing dilute sulfuric acid pretreatment and post treatment. Fuel 275, 117943 (2020).
Vanyan, L., Cenian, A. & Trchounian, K. Biogas and biohydrogen production using spent coffee grounds and alcohol production waste. Energies 15, 5935 (2022).
Dareioti, M. A., Tsigkou, K., Vavouraki, A. I. & Kornaros, M. Hydrogen and methane production from anaerobic co-digestion of sorghum and cow manure: Effect of pH and hydraulic retention time. Fermentation 8, 304 (2022).
Yin, D.-M., Mahboubi, A., Wainaina, S., Qiao, W. & Taherzadeh, M. J. The effect of mono-and multiple fermentation parameters on volatile fatty acids (VFAs) production from chicken manure via anaerobic digestion. Bioresour. Technol. 330, 124992 (2021).
Article CAS PubMed Google Scholar
Yin, D. M. et al. Volatile fatty acids (VFA) production and recovery from chicken manure using a high-solid anaerobic membrane bioreactor (AnMBR). Membranes 12, 1133 (2022).
Article CAS PubMed PubMed Central Google Scholar
Xiang, C. et al. Why can hydrothermally pretreating lignocellulose in low severities improve anaerobic digestion performances?. Sci. Total Environ. 752, 141929 (2021).
Article CAS PubMed Google Scholar
Yuan, H. et al. Effect of low severity hydrothermal pretreatment on anaerobic digestion performance of corn stover. Bioresour. Technol. 294, 122238 (2019).
Article CAS PubMed Google Scholar
Bondesson, P.-M., Galbe, M. & Zacchi, G. Ethanol and biogas production after steam pretreatment of corn stover with or without the addition of sulphuric acid. Biotechnol. Biofuels 6, 1–11 (2013).
We want to express our sincere gratitude to the University of Borås and Amirkabir University of Technology for their support and resources that have contributed significantly to the completion of this research project.
Department of Chemical Engineering, Amirkabir University of Technology, Tehran, 15875-4413, Iran
Sepideh Oladzad, Narges Fallah, Neda Afsham & Javad Toghyani
Swedish Center for Resource Recovery, The University of Borås, 501 90, Borås, Sweden
Amir Mahboubi & Mohammad J. Taherzadeh
You can also search for this author in PubMed Google Scholar
You can also search for this author in PubMed Google Scholar
You can also search for this author in PubMed Google Scholar
You can also search for this author in PubMed Google Scholar
You can also search for this author in PubMed Google Scholar
You can also search for this author in PubMed Google Scholar
The conception and design of the study were contributed to by Sepideh Oladzad, Narges Fallah, Amir Mahboubi and Mohammad J. Taherzadeh. Sepideh Oladzad was in charge of conceptualization, research, and data preparation. Javad Toghyani and Sepideh Oladzad carried out the formal analysis. Sepideh Oladzad wrote the manuscript’s initial draft. Narges Fallah, Amir Mahboubi, Mohammad J. Taherzadeh, and Neda Afsham worked on the manuscript’s editing and revision. Narges Fallah, Amir Mahboubi, and Mohammad J. Taherzadeh handled the funding procurement and supervision. The final manuscript was read and approved by all of the authors.
The authors declare no competing interests.
Springer Nature remains neutral with regard to jurisdictional claims in published maps and institutional affiliations.
Open Access This article is licensed under a Creative Commons Attribution-NonCommercial-NoDerivatives 4.0 International License, which permits any non-commercial use, sharing, distribution and reproduction in any medium or format, as long as you give appropriate credit to the original author(s) and the source, provide a link to the Creative Commons licence, and indicate if you modified the licensed material. You do not have permission under this licence to share adapted material derived from this article or parts of it. The images or other third party material in this article are included in the article’s Creative Commons licence, unless indicated otherwise in a credit line to the material. If material is not included in the article’s Creative Commons licence and your intended use is not permitted by statutory regulation or exceeds the permitted use, you will need to obtain permission directly from the copyright holder. To view a copy of this licence, visit http://creativecommons.org/licenses/by-nc-nd/4.0/.
Oladzad, S., Fallah, N., Mahboubi, A. et al. Comparison of acid and hydrothermal pretreatments of date waste for value creation. Sci Rep 14, 18056 (2024). https://doi.org/10.1038/s41598-024-68879-6
DOI: https://doi.org/10.1038/s41598-024-68879-6
Anyone you share the following link with will be able to read this content:
Sorry, a shareable link is not currently available for this article.
Provided by the Springer Nature SharedIt content-sharing initiative
Scientific Reports (Sci Rep) ISSN 2045-2322 (online)

Acetic Acid Glacial Sign up for the Nature Briefing: Anthropocene newsletter — what matters in anthropocene research, free to your inbox weekly.