Diode lasers are divided into two broad categories depending on the wavelengths they generate. Short-wavelength diode lasers produce light at visible and very-near-infrared wavelengths (below 1 µm), while long-wavelength diode lasers emit from 1 µm to well into the mid-infrared range— up to 12 µm in some experimental models. Within the long-wavelength category, fiberoptic-communications lasers operate in the near-infrared low-attenuation windows of silica glass fibers at 1.3 and 1.51 µm (see photo at top of this page). The broad range of mid-infrared diode lasers is mainly used for detecting gases, including, in particular, air pollutants, through their infrared (IR) absorption bands. The rapid expansion of diode lasers into ever longer-wavelength regions is a major area of laser research today.
In the design of communications lasers, speed of response is of the essence— a laser must be able to respond rapidly to an imposed modulating signal that may be at a frequency of tens of gigahertz. The key to achieving such rapid response is the reduction of parasitic capacitance. There are a number of structures that accomplish this goal and in addition are relatively easy to manufacture. Surgical Laser
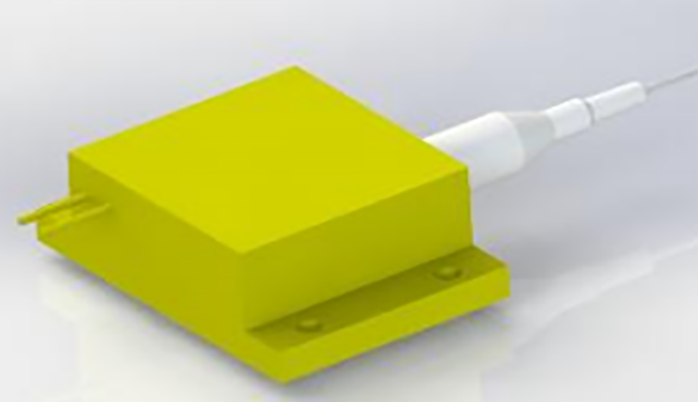
Long-term reliability is equally important in communications lasers. Certain key failure modes limit laser performance and lifetime. First, catastrophic failure due to melting can occur with power densities at the output facet above 1 mW/cm2, which corresponds to powers of a few tens of milliwatts for facets with a cross section of a few square microns. Facet coatings such as alumina can increase these levels significantly.
Second, dark-line defects can be generated by the accumulation of a network of dislocations. Such defects are regions of strong nonradiative recombination of electrons and holes and may start from a single dislocation. Because they sap the efficiency of the laser, they lead to rapid decrease in output power and device failure. Their elimination depends on the use of dislocation-free substrates (those with fewer than ten dislocations per square millimeter). Dislocation can also be generated during soldering of contact wires, so high temperatures and mechanical pressures must be minimized. In addition, lasers are screened through a burn-in period that eliminates devices with early dark-line defects.
Even if dark-line defects are eliminated, there is a gradual decrease in laser power and an increase in threshold current with time as nonradiative recombination releases energy that forms dislocations, leading to more nonradiative recombination. However, typical diode lasers have mean lifetimes on the order of 100 years.
While any diode laser produces a narrow range of wavelengths and quantum-well lasers, described below, produce even narrower lines with widths of a few nanometers (or a few tenths of a percent of the carrier wavelength), even smaller linewidths, around 0.05 nm, are required for fiberoptic communications to minimize dispersion. In addition, the spread of frequencies in the carrier should not exceed 10% of the bit rates, so even for gigabit rates, this requires a line width of 100 MHz, or about 0.001 nm.
The applications for diode lasers in the mid-IR are far more limited than the huge telecommunications market but are nonetheless important. In this spectral range, lasers can be used to detect gases either for environmental monitoring or for industrial-process control.
The problem in obtaining such long wavelengths is that few suitable materials have bandgaps small enough to generate mid-IR radiation. One of the most promising approaches to overcoming this problem is using periodically poled lithium niobate (LiNbO3) to increase the wavelength of light from optical diode lasers. This technique is the inverse of the familiar frequency-doubling crystals used to produce short-wavelength laser light.
In one scheme, two tunable lasers are fed into a LiNbO3 crystal, one in the range about 0.98 µm, the other around 0.78 µm. In the crystal, the two waves interact nonlinearly to produce the difference frequency, with wavelengths in the range between 3.6 and 4.3 µm at power levels of 7 µW. In these nonlinear crystals, for reasonable efficiencies, the different wavelengths must remain phase-matched, which is not possible in a material where the refractive index changes with wavelength, because the different wavelengths travel at different velocities. In periodically poled lithium niobate (PPLN), this problem is overcome by periodically reversing the ferroelectric domain structure and thus the direction of spontaneous polarization. If this is done with a period equal to the coherence length of the nonlinear infraction, phase-matching is maintained.
There are other ways of modifying the wavelength of light to produce mid-IR radiation, especially in the range beyond 5 µm where absorption cuts off the use of LiNbO3. The most important is the quantum-well structure, which also improves the general operation of a diode laser. When a layer of material in a semiconductor is made sufficiently thin, less than 50 nm, quantum effects become important because the location of the electrons is so restricted in one dimension. The result is that the conduction band and valence band split into sub-bands, and the probability of a transition across the bandgap also changes.
An important modification of the quantum-well structure is the quantum cascade. In this structure, the transitions are not between the valence and conduction bands but between two conduction bands. The close spacing of these bands allows such lasers to emit light in the mid- to far-IR range. A number of research groups are working to push quantum-cascade wavelengths to longer wavelengths. At Lucent Technologies – Bell Labs (Murray Hill, NJ), Jerome Faist and colleagues have achieved 60 mW of power at room temperature at 5.4 and 8 µm. The device uses alternating layers of AlInAs and GaIn with a distributed-feedback structure. More recently, the same group pushed wavelengths up to 11.2 µm with the device cooled to 200 K. There does not appear to be any inherent limit in how long a wavelength can be produced with the quantum-cascade structure.
Jerome Faist et al., "Continuous wave Quantum Cascade Lasers," SPIE vol. 2682, p. 198 (May 1996).
G. J. Dixon, Laser Focus World 33(5), 105 (May 1997).
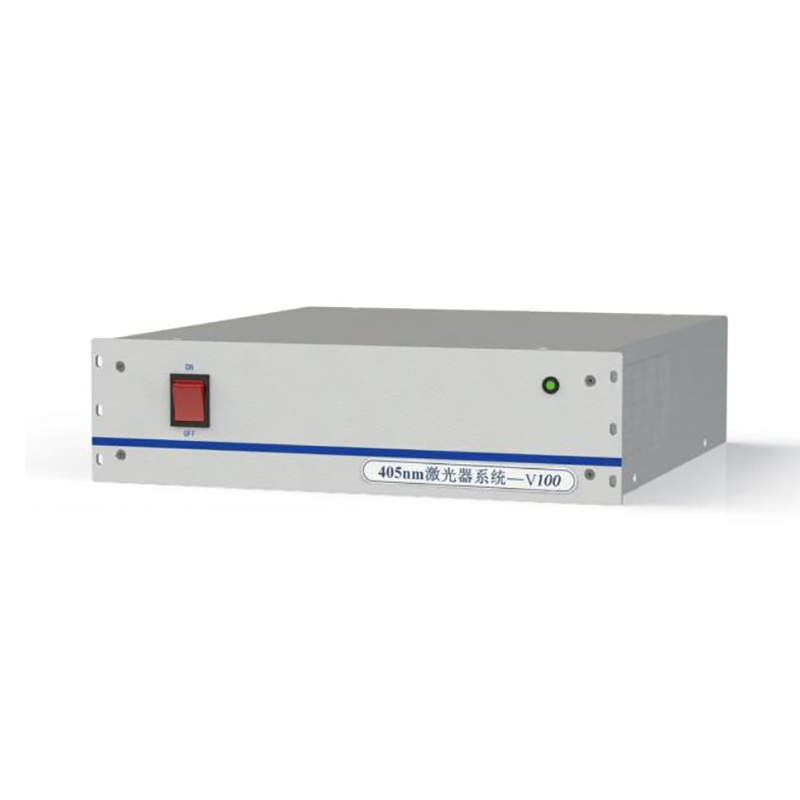
Uv Laser Diode Eric J. Lerner is a contributing editor for Laser Focus World.