Starting out with a motorcycle permit just because he could get one two years earlier than a driver's license, Mircea keeps his passion for bikes (motor or no motor) alive to this day. His lifelong dream is to build his own custom camper van. Full profile
You will only receive our top stories Prisms

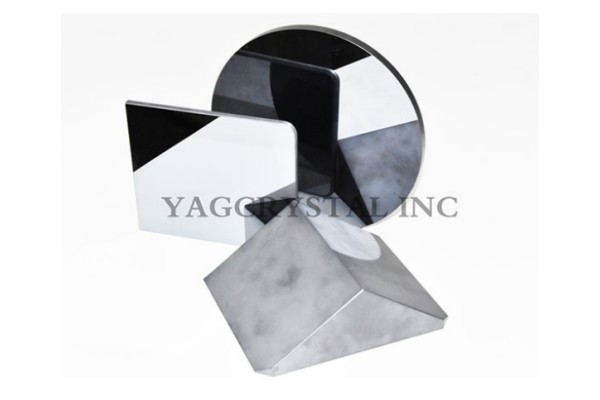
Bilateral Yag © 2008-2023 SoftNews Net SRL • Privacy Policy • Cookies Policy • Terms of Use All rights reserved. autoevolution® and the autoevolution® logo are registered trademarks.