Thank you for visiting nature.com. You are using a browser version with limited support for CSS. To obtain the best experience, we recommend you use a more up to date browser (or turn off compatibility mode in Internet Explorer). In the meantime, to ensure continued support, we are displaying the site without styles and JavaScript.
Scientific Reports volume 14, Article number: 26819 (2024 ) Cite this article Ems Muscle Stimulator
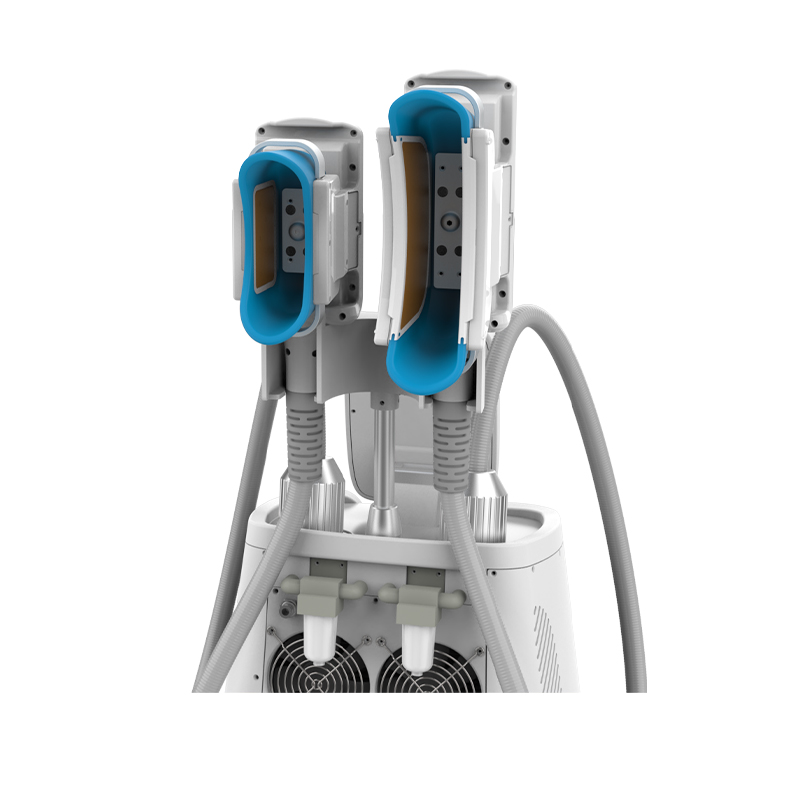
Sperm motility is a crucial factor in male fertility. Photobiomodulation (PBM) has been reported to increase sperm motility, but a consistent approach suitable for identifying standardizable protocols is lacking. We collected asthenozoospermic (n = 70) and normozoospermic (n = 20) semen. The asthenozoospermic samples were irradiated with an 810 nm diode laser, in continuous wave mode, at 0.25 W, 0.5 W, 1 W and 2 W for 60 s on a circular area of 1 cm2 through a novel handpiece with an innovative flat-top profile. Sperm motility was assessed immediately, after 30 and 60 min. A sample size calculator, unpaired t-test and one-way ANOVA with post-hoc Tukey HSD tests were used for statistics. One and 2 W were the most effective outputs in increasing progressive motility compared to control (p < 0.001). The maximum effect was immediately after 1 W-PBM (p < 0.001) and decreased after 60 min (p < 0.001). Time physiologically decreased vitality (p < 0.001), but less in the 1 W-PBM samples (p < 0.05). 1 W-PBM did not affect chromatin condensation. Asthenozoospermic samples displayed an impairment of 80% in oxygen consumption and ATP production and a slight inefficiency of oxidative phosphorylation compared to normozoospermic samples (p < 0.001). 1 W-PBM partially restored the functionality of aerobic metabolism (p < 0.001) by recovery of oxidative phosphorylation efficiency. PBM did not affect lactate dehydrogenase (glycolysis pathway). No irradiated samples increased accumulated malondialdehyde, a marker of lipidic peroxidation. In conclusion, PBM improves progressive motility in asthenozoospermia through increased mitochondrial energetic metabolism without harmful oxidative stress.
Infertility is a global public health problem that affects about 10% of couples. Approximately 50% of cases involve a male factor alone (30%) or combined with a female factor (20%)1. The prevalence of male infertility is steadily increasing worldwide as semen quality and other markers of male reproductive health have declined considerably over the past decade2. Sperm motility is a crucial parameter in male fertility, and it is affected by energy consumption3.
Mature spermatozoa consist of a head with a nucleus, a neck, and a flagellum that allows motility. It comprises three parts; mitochondria are present only in the midpiece4. They are tightly interconnected and helically wound around the axoneme, forming the mitochondrial sheath. In humans, 10–12 mitochondrial cores are formed by 72–80 oblong mitochondria5. The primary function of mitochondria is to produce energy. Mammalian sperm uses ATP for motility, capacitation, hyperactivation, acrosomal reaction, and oocyte penetration. However, there is still debate about the metabolic pathways responsible for energy production in spermatozoa4,6. Indeed, oxidative phosphorylation (OxPhos) and anaerobic glycolysis are not mutually exclusive, and sperms can use both pathways. Makai and Travis7 have suggested that ATP production in the mitochondrial membrane may not be sufficient for diffusion throughout the flagellum. It has been postulated that mitochondria produce ATP through OxPhos in the midpiece and that anaerobic glycolysis provides ATP in the midpiece. Piomboni et al.8 reviewed the literature on mitochondrial energy production and its role in human sperm motility and found a link between structural abnormalities and impaired sperm mitochondrial function in asthenozoospermia.
The possibility of energizing mitochondria through the irradiation of visible or near-infrared light has attracted increasing interest over the last 40 years, starting with the first evidence suggested by Karu et al.9,10 and later by Passarella et al.11 with a wavelength of about 630 nm. Recently, the possibility of stimulating photoacceptors in mitochondrial complexes with different wavelengths in the near-infrared has been characterized. All the research showed increased ATP production through the photo-energization of cytochromes. In particular, a diode laser light of 810–980 nm mainly modulated complex IV and, to a lesser extent, III, while 1064 nm stimulated complexes I, III, and IV12,13,14,15,16. Medical and veterinary use of this phototherapy is known as photobiomodulation (PBM), formerly referred to as low-level laser therapy. It is used to support recovery from various diseases often associated with mitochondrial dysfunction17,18,19,20,21.
Reports on animals suggest that PBM may improve spermatogenesis22,23,24 or increase sperm motility25,26,27,28,29,30,31 in fish, mice, dogs, boar, ram, and cattle models.
Several studies conducted in vitro on human spermatozoa highlighted the potential benefits of PBM on sperm function20,32,33,34,35. Almost all studies have examined the positive impact of PBM therapy on the increase in the motility of human sperm from healthy subjects36,37,38,39,40,41,42. Some studies included sperm from oligozoospermic and asthenozoospermic patients and showed that irradiation significantly increased sperm motility also in abnormal sperm37,39,42,43,44. In two studies, PBM therapy before cryopreservation, even in normozoospermic semen samples, played a protective role against the detrimental effects of cryopreservation by preserving the functional parameters of spermatozoa45,46.
Overall, as often happens in the field of PBM therapy, standardization of application protocols remains a challenge due to the heterogeneity of population characteristics and sample size, the type of intervention, and the different LED/laser light parameters (in terms of wavelength, output power, distance, energy density, and other characteristics of the radiation probes), duration of exposure and manifestation of effects. In addition, the biological mechanisms of PBM on sperm are not fully known, and limited safety assessments are available, sometimes with conflicting data. For instance, Lenzi et al.36 have shown that an increase in progressive sperm motility after laser irradiation (647 nm) was associated with a faster rate of sperm ATP consumption, suggesting that laser irradiation may have an “energy modulation effect” on normal sperm. However, later, it was observed that near-infrared radiation resulted in a loss of cell viability, increased lipid peroxidation leading to diminished membrane function, and higher susceptibility of sperm DNA to fragmentation, resulting in induction of apoptosis47.
Our work investigated how PBM with 810 nm wavelength irradiated at 0.25 W, 0.5 W, 1 W and 2 W for 60 s on a circular area of 1 cm2 impacts the sperm of men with asthenozoospermia. The selected parameters were delivered using a new handpiece with a flat irradiation profile (FT-HP). In a previous work, we have described and characterised this handpiece as having improved performance compared to conventional fibres or standard Gaussian profiled handpieces48,49. To validate an optimised protocol, we first identified which of the chosen irradiation parameters could maximise the performance of asthenospermic spermatozoa. Then, we analyzed the impact of PBM on sperm motility and its duration after irradiation. The effectiveness of photobiomodulation on sperm energy metabolism was tested by monitoring OxPhos and lactate dehydrogenase (LDH), which is involved in the glycolytic energy pathway. Photobiomodulation safety was assessed by analyzing sperm vitality, DNA fragmentation, and lipidic peroxidation.
The irradiations were done with the ENEA GaAl-As diode laser model (Garda Laser S.A.S., Verona, Italy). The device allowed irradiating at a wavelength of 810 nm ± 2 for 60 s in continuous wave mode. The irradiation power was set at 0.25, 0.5, 1.0, or 2.0 W to generate an energy of 15.0, 30.0, 60.0, or 120.0 J (power density of 0.25, 0.5, 1.0, or 2.0 W/cm2; fluence of 15.0, 30.0, 60.0–120.0 J/cm2). The control samples’ power was set to 0.0 W. Irradiations were delivered using a novel FT-HP, which can improve irradiation performance compared to conventional fibres and standard Gaussian profiled handpieces. Indeed, our previous characterization of the FT-HP showed that it can provide a uniform and consistent energy distribution over a spot area of 1 cm2, which is independent by distance48,49. A 635 nm red light pointer (negligible power, < 0.5 mW) was used in both treatments to visualize the exposed area and maintain experimental blinding. A Pronto-250 power meter (Gentec Electro-Optics, Inc. G2E Quebec City, Canada) was used to ensure the accuracy of the irradiated laser parameters. The irradiations were carried out with the handpiece fixed to a stand and in contact mode with the surface of the multiwells plate (diameter of a single well approximately 1.1 cm). All irradiations were performed with the multiwells plate placed on a Metal Velvet light-absorbing plate (Acktar Ltd., 8643 Kiryat-Gat, Israel). The extremely low reflectance of the plates, which is guaranteed to be equal to or less than 1% in the near-infrared band, significantly reduces reflections from the top of the bench. Any undesirable thermal effects were avoided by monitoring the exposure with a FLIR ONE Pro-iOS thermal camera (FLIR Systems, Inc., Portland, OR, USA) (dynamic range: -20 °C/+400 °C; resolution 0.1 °C).
We collected semen samples from donors undergoing semen analysis for fertility diagnosis at the SS Physiopathology of Human Reproduction, IRCCS Ospedale Policlinico San Martino, Genova, Italy, from December 2023 to May 2024. The study complied with the Declaration of Helsinki50 and was approved by the Ethics Committee of Regione Liguria (219/2024 - DB id 13852). Written informed consent was obtained from all donors, and participation was voluntary. For the basic examination of semen samples, the following parameters were checked based on WHO criteria51: semen volume, sperm count per milliliter, sperm motility, and percentage of sperm with normal morphology. Inclusion criteria: normozoospermia for control samples with ≥ 30% progressive motility, according to the WHO criteria. Based on the same criteria, asthenozoospermia for irradiated samples was defined as < 42% total motility, < 30% progressive motility. Exclusion criteria: samples with < 1.5 ml semen volume, semen with sperm agglutinations, round cells, bacterial contamination, severe oligospermia (< 10 million sperms per ml, < 35 million sperms per ejaculate), genetic syndrome. Sperm morphology was not included among the inclusion/exclusion criteria since we focused on the effects of laser irradiation on sperm motility.
PBM therapy for sperm was carried out in native ejaculates. Each asthenozoospermic sample was divided into 5 equal aliquots (200 µl each). Four out of 5 were irradiated at different powers (0.25 W, 0.5 W, 1 W, 2 W), respectively. One aliquot served as a control and received laser treatment with 0.0 W. A second operator trained in laser therapy then treated the samples. Sperm motility was assessed in a blinded manner immediately after irradiation (T0), after 30 (T30) and 60 min (T60) by an experienced operator. Sperm chromatin dispersion and membrane integrity were assessed blindly in untreated and 1 W irradiated asthenozoospermic samples after 60 min. Energetic metabolism, oxidative stress, and oxidative damage were analyzed in normozoospermic samples as a reference for analyzing metabolism and control and 1 W irradiated asthenozoospermic samples. Figure 1 summarizes the experimental setup.
Study design. The patients were recruited at the SS Physiopathology of Human Reproduction, IRCCS Ospedale Policlinico San Martino, Genova, Italy (A). Semen samples were analyzed (B), and according to the inclusion and exclusion criteria, 70 astenozoospermic semen and 20 normozoospermic semen samples were included in our study (C). Astenozoospermic samples were divided into aliquots and irradiated with 810 nm photobiomodulation therapies (D). Samples irradiated with laser switch-off were considered controls. To assess the beneficial or detrimental effects of the treatment, the asthenozoospermic samples were then analyzed in a blinded fashion (E). The normozoospermic samples’ energy metabolism and oxidative stress were also examined and considered (E).
All individuals collected semen by masturbation into a sterile container after 2 to 5 days of abstinence. Sperm samples were liquefied at room temperature for 30–60 min.
Sperm count and motility were assessed using a disposable counting chamber (AB Cell-VU, AB Analitica, Padova, Italy). It consists of a standard specially-designed glass slide and a coverslip with the counting grid laser-etched into its surface. The 1 × 1 mm grid is divided into 100 squares, each one of 0.1 × 0.1 mm, and the chamber has a depth of 20 μm. This depth is optimal for sperm cells to form in a monolayer, so motility can be assessed and counts are made easily. Briefly, after mixing the undiluted sample thoroughly, one drop (approximately 5 µl) of semen was placed at the sampling area of the slide and the coverslip was gently lower over the specimen, avoiding air bubbles on the counting area. Sperm concentration was determined by counting all motile and non-motile sperm within a row of 10 squares, that is one millionth of ml. Therefore, the number of sperm heads in 10 squares indicates their concentration in millions/ml.
The number of motile sperms within 10 squares of the grid was divided by the total sperm (motile + non-motile). The multiplication of this number by 100 established the percentage of motile sperm.
The sperm score for motility evaluation was expressed as follows: (a) rapid progressive motility refers to sperm that are swimming fast in a mostly straight line; (b) slow progressive motility refers to sperm that are swimming slowly in large circles; (c) non-progressive motility refers to sperm that swim in situ; (d) immobility refers to non-motile sperm. The progressive motility rate was calculated as the percentage of a + b.
To check for the safety of the sperm-PBM treatment, sperm vitality was estimated 60 min after irradiation by assessing the membrane integrity of the cells by dye exclusion (dead cells have damaged plasma membranes that allow entry of membrane-impermeant stains). Briefly, a 50-µl aliquot of semen was mixed with an equal volume of eosin (Life test, AB Analitica, Italy); after 30 s, it was smeared on a glass slide and immediately examined under an Eclipse Si microscope (Nikon Europe B.V., Amstelveen, The Netherlands) at 20X magnification. Spermatozoa with red or dark pink heads were considered dead, whereas spermatozoa with white heads were considered alive. A minimum of 200 sperms per sample was counted, and the percentage of live sperms was calculated.
For further confirmation of the safety of PBM treatment, measurement of sperm DNA fragmentation was performed 60 min after irradiation. To provide results as repeatable and consistent as possible we used a commercial kit (Halosperm G2 kit, Halotech DNA SL, Madrid, Spain) that is based on the Sperm Chromatin Dispersion (SCD) technique. Fresh sperms are immersed in an inert agarose microgel on a pretreated slide. An initial acid treatment denatures DNA in those sperms with fragmented DNA. Following this, a controlled DNA denaturation process facilitates the subsequent removal of most nuclear proteins in each spermatozoon. In this way, normal spermatozoa create halos formed by loops of DNA at the head of the sperm, which are not present in those with damaged DNA. When no massive DNA breakage is present, nucleoids from sperm with fragmented DNA either do not show a dispersion halo or the halo is minimal. Spermatozoa without DNA fragmentation show a dispersion halo and spermatozoa with fragmented DNA do not show a dispersion halo, or the halo is minimal. A minimum of 300 sperms per sample were counted using conventional microscopy (Eclipse Si, Nikon) at 20X magnification, and the percentage of sperms with fragmented DNA was calculated. Values of DNA fragmentation above 30% were considered pathological.
The OxPhos function, in terms of ATP synthesis and oxygen consumption rate (OCR), was assessed in 50 µg of total protein of normozoospermic samples, asthenozoospermic samples, PBM-treated asthenozoospermic samples immediately or after 30 min from the laser administration permeabilized with 0.01% digitonin and resuspended in phosphate-buffered saline (PBS).
ATP synthesis was measured by a GloMax 20/20 Luminometer (Promega, Milan, Italy) at 30-second intervals over 2 min using the luciferin/luciferase methods. OCR was measured using an amperometric electrode (Unisense Microrespiration, Unisense A/S, Aarhus, Denmark) in a sealed chamber. For both assays, samples were resuspended in PBS, and 10 mM pyruvate and 5 mM malate were used as respiring substrates and 0.1 mM ADP was added just before the start of the evaluations52.
To assess the OxPhos efficiency the P/O value was calculated as the ratio between the produced ATP and the consumed oxygen. Values around 2.5 indicate a whole coupling between energy synthesis and respiration; lower values indicate an uncoupling status53,54.
The energy status was evaluated as the ratio between ATP and AMP intracellular concentrations. ATP and AMP intracellular concentrations were quantified using the enzyme coupling method, employing 50 µg of total protein for each assay. ATP measurement involved monitoring NADP reduction at 340 nm. The assay medium contained 50 mM Tris-HCl pH 8.0, 1 mM NADP, 10 mM MgCl2, and 5 mM glucose. Samples were analyzed spectrophotometrically before and after the addition of 4 µg of purified hexokinase plus glucose 6-phosphate dehydrogenase55. AMP level was evaluated following the NADH oxidation at 340 nm, using an assay solution composed by 100 mM Tris-HCl pH 8.0, 75 mM KCl, 5 mM MgCl2, 0.2 mM ATP, 0.5 mM phosphoenolpyruvate, 0.2 mM NADH, 10 IU adenylate kinase, 25 IU pyruvate kinase, and 15 IU of lactate dehydrogenase55.
Lactate dehydrogenase (LDH; EC 1.1.1.27) activity was assessed using 20 µg of total protein by tracking NADH oxidation at 340 nm with a double-beam spectrophotometer (UNICAM UV2, Analytical S.n.c, Langhirano, Italy). The reaction mixture consisted of 100 mM Tris-HCl pH 7.4, 0.2 mM NADH, and 5 mM pyruvate56. The assay started with the sample addition and was monitored for at least five minutes every 1 min.
Malondialdehyde (MDA) concentration was determined as a marker of lipid peroxidation to evaluate oxidative damage using the thiobarbituric acid reactive substances (TBARS) assay. The TBARS solution contains 15% trichloroacetic acid in 0.25 N HCl and 26 mM thiobarbituric acid. To evaluate the basal concentration of MDA, 600 µl of TBARS solution was added to 50 µg of total protein dissolved in 300 µl of milliQ water. The mix was incubated for 40 min at 95 °C. Then the sample was centrifuged at 14,000 rpm for 2 min and the supernatant was analyzed spectrophotometrically at 532 nm56.
According to Rosner57, the sample size for the PBM therapy screening was calculated using the sample size calculator available at ClinCalc.com. Based on the preliminary data and considering a beta of 0.2, an alpha significance level of 0.05, and a power of 0.8 for the initial screening trials with the 0.25, 0.5, 1, and 2 W powers, a minimum of 18 patients was considered necessary to detect a clinically significant difference. One-way analysis of variance (ANOVA) was used to analyze the data. Tukey’s multiple comparison test was then performed. The software used was Prism 8 (GraphPad software, Boston (MA), USA). The comparison between the means of two independent samples was performed using an unpaired t-test. The data are presented as the mean ± standard deviation (SD). The statistical significance was determined at a probability level of p < 0.05.
Progressive motility screening (0.25-0.5-1–2 W) was performed in 20 asthenozoospermic patients according to the results of the sample size calculation. Afterwards, the study was conducted on a total of 70 asthenozoospermic patients using 810 nm PBM therapy at 1 W power. In the energy metabolism and oxidative stress studies, semen from 20 normozoospermic patients was used as a baseline for comparison. Overall, the cohort consisted of 100% Caucasian males, aged 34.1 ± 8.8 years for the asthenozoospermic patients and 35.1 ± 9.4 years for the normozoospermic patients, with no significant difference between groups. In May 2024, patient recruitment and sample analysis were completed.
All treated samples exhibited impaired sperm motility, with less than 30% initial progressive motility according to WHO criteria51. Impaired non-irradiated samples were used for comparison. Figure 2 reports the effect of an 810 nm laser on asthenozoospermic sperm progressive motility immediately after irradiation at different powers (0.25-0.5-1-2 W). The results show that only 1 W and 2 W significantly (p<0.01) increased progressive motility relative to the control, inducing an increment of 50%.
Graph showing results of progressive motility in samples of 20 asthenozoospermic patients immediately after irradiation at different powers. The progressive motility rate was calculated as the percentage of a + b, where a corresponds to rapid progressive motility and b to slow progressive motility. Data are expressed as mean ± SD and compared with one-way ANOVA followed by Tukey’s multiple comparison test. ** indicates a significant difference (p < 0.01) compared to the control.
Based on the above screening protocol results, 1 W and 2 W have been identified as the laser power capable of maximizing sperm motility parameters. Further experiments were performed at 1 W, which we previously demonstrated to be effective and safe in an urchin sea in vitro model58. PBM improved progressive motility according to the post-exposure time: the maximum effect with respect to control was obtained immediately after irradiation (T0) (29% versus 36%, p < 0.001) and kept high within 30 min (T30) (29% versus 35%, p < 0.01) with a decrease after 60 min (T60) (27% versus 31%, p > 0.05) (Fig. 3A).
Compared to controls, the immotile fraction of sperms significantly reduced with an average percentage decrease of -11% at T0 and T30 (p< 0.05) and -9% at T60 (p> 0.05) (Fig. 3B).
Bar graphs showing PBM effects on sperm motility of 70 asthenozoospermic patients. (A) percentage of progressive motility, and (B) percentage of immotile sperm in control and treated samples over time (T0: immediately after irradiation; T30: 30 min after irradiation; T60: 60 min after irradiation). Data are expressed as mean ± SD. Comparisons were made by one-way ANOVA followed by Tukey’s multiple comparison test. *, **, and *** indicate a significant difference for p < 0.05, p < 0.01, and p < 0.001, respectively, between the control and the treated sample at the same time point.
Figure 4A shows the presence of viable and nonviable sperm samples from 25 asthenozoospermic patients after eosin staining. Figure 4B shows the viability test results on the samples at the baseline and controls and the irradiated samples at 60 minutes after irradiation. Time physiologically decreased vitality with respect to the baseline (control T0) (p<0.001). However, the process is less evident in the PBM samples (laser T60) than in the control T60 (p<0.05).
Sperm viability test using eosin staining. (A) A representative image of sperm viability test. Live sperm heads were unstained, and dead sperm heads were stained red-pink (bar: 25 μm). (B) Graph showing the viability percentage at the baseline (control T0) and after 60 min from irradiation in controls (control T60) and treated samples (laser T60) of 25 asthenozoospermic patients. Data were expressed as mean ± SD and compared with one-way ANOVA followed by Tukey’s multiple comparison test. *** indicates a significant (p < 0.001) difference between control T0 vs. control T60 and laser T60, respectively. # indicates a significant (p < 0.05) difference between control T60 vs. laser T60.
Our analysis showed that chromatin dispersion in sperm samples from 25 asthenozoospermic patients remained stable after 60 minutes from PBM (p>0.05) (Fig. 5). We did not detect significant differences in the DNA fragmentation level after PBM compared to untreated sperm. Interestingly, even 5 samples with a positive SCD test (>30%) at the baseline (41.2% ± 5.0%) did not display an increased value of chromatin dispersion after irradiation (41.1% ± 5.1%).
Sperm DNA integrity test using Sperm Chromatin Dispersion (SCD) technique. (A) A representative image of sperm DNA integrity test. Sperm without DNA fragmentation shows a dispersion halo, sperm with fragmented DNA do not show a dispersion halo, or the halo is minimal (bar: 50 μm). (B) The percentage of sperm with fragmented DNA after 60 min from irradiation in controls (control T60) and treated samples (laser T60) of 25 asthenozoospermic patients. Data reported in Panel B were expressed as mean ± SD and compared with the unpaired t-test.
PBM effect on OxPhos activity in samples from 20 asthenozoospermic and 20 normozoospermic patients. (A) ATP synthase activity; (B) oxygen consumption rate; (C) P/O ratio as a marker of OxPhos efficiency in normozoospermic samples (normo), asthenozoospermic samples (astheno) and asthenozoospermic samples lasered and immediately evaluated (astheno + laser T0) or evaluated after 30 min (astheno + laser T 30). Data are expressed as mean ± SD. Statistical analysis was performed using one-way ANOVA followed by Tukey’s multiple comparison test. *** indicates a significant difference (p < 0.001) between normo and astheno, astheno + laser T0), or astheno + laser T 30. ### indicates a significant difference (p < 0.001) between astheno versus astheno + laser T0 or astheno + laser T30.
To verify whether the increased motility observed in asthenozoospermic samples after PBM could be due to an enhancement in cellular energy metabolism, the OxPhos function was assessed by comparing the results obtained in samples from 20 asthenozoospermic and 20 normozoospermic patients. The data reported in Fig. 6 show that, under basal conditions, asthenozoospermic samples produce less ATP due to reduced oxygen consumption than normozoospermic samples, suggesting a deficit in OxPhos. This decrease only regards energy yield, as mitochondrial efficiency, indicated by the P/O ratio, appears similar to that observed in normozoospermic samples. The reduction in aerobic metabolism in asthenozoospermic samples is partially reversed after treatment with PBM, as both ATP synthesis and OCR increase by approximately 50% compared to the untreated sample immediately after irradiation and in the following 30 min. This increase does not alter the coupling between chemical energy synthesis and cellular respiration, as P/O values remain consistently around 2.5.
PBM-induced changes of cellular energy status in samples from 20 asthenozoospermic and 20 normozoospermic patients. (A) ATP intracellular concentration; (B); AMP intracellular concentration; (C) ATP/AMP ratio as a marker of cellular energy status in normozoospermic samples (normo), asthenozoospermic samples (astheno) and asthenozoospermic samples lasered and immediately evaluated (astheno+laser T0) or evaluated after 30 minutes (astheno+laser T 30). Data are expressed as mean + SD. Statistical analysis was performed using one-way ANOVA followed by Tukey’s multiple comparison test. *** indicates a significant difference (p < 0.001) between normo and astheno, astheno+laser T0), or astheno+laser T 30. ### indicates a significant difference (p<0.001) between astheno versus astheno+laser T0 or astheno+laser T30.
Following the increase in OxPhos, it was examined whether the cellular energy state also increased by evaluating the ATP and AMP intracellular concentrations and their ratio (Fig. 7). Samples from 20 asthenozoospermic and 20 normozoospermic patients were analyzed. The data show that asthenozoospermic samples are characterized by low ATP levels and high AMP concentration compared to normozoospermic samples. This results in a low cellular energy state that could explain the reduced motility. The increase in OxPhos in asthenozoospermic samples induced by PBM causes a 1.5-fold increase in intracellular ATP concentration and a reduction of about 50% in AMP levels, leading to a 3.5-fold increase in the ATP/AMP ratio. This effect is observed both immediately after irradiation and 30 min post-treatment. However, it is a reversible effect, as 60 min after laser administration, ATP, AMP levels, and the ATP/AMP ratio return to values similar to those of untreated asthenozoospermic samples. This finding is not surprising, as it has been demonstrated that the effect of PBM on isolated mitochondria is reversible59 However, the increase in the energy state of asthenozoospermic samples could ensure improved motility for a sufficient time to allow fertilization.
Usually, if OxPhos is defective, cells attempt to compensate for the lack of ATP synthesis and the conversion of NADH to NAD+ by increasing anaerobic glycolysis. However, samples from 20 asthenozoospermic patients do not seem to employ this strategy, as the levels of lactate dehydrogenase (LDH), the enzyme that catalyzes the conversion of pyruvate to lactate, are not higher than those in samples from 20 normozoospermic patients, nor does PBM treatment alter its activity (Fig. 8).
PBM effect on LDH activity in samples from 20 asthenozoospermic and 20 normozoospermic patients. Graph reports the LDH activity in normozoospermic samples (normo), asthenozoospermic samples (astheno), and asthenozoospermic samples lasered and immediately evaluated (astheno+laser T0) or assessed after 30 minutes (astheno+laser T 30). Data are expressed as mean + SD. Statistical analysis was performed using one-way ANOVA followed by Tukey’s multiple comparison test, and no significant differences were observed.
Considering that aerobic metabolism is always associated with the production of oxidative stress, even under conditions of complete coupling between ATP synthesis and respiration, the concentration of malondialdehyde (MDA), a marker of lipid peroxidation, was assessed. Samples from 20 asthenozoospermic and 20 normozoospermic patients were analyzed. The data shown in Fig. 9 indicate that asthenozoospermic samples under basal conditions display a higher concentration of MDA compared to normozoospermic samples. However, the MDA level does not increase following PBM treatment, suggesting that the increase in asthenozoospermic samples OxPhos does not induce further oxidative damage.
PBM effects on lipid peroxidation accumulation were evaluated in samples from 20 asthenozoospermic and 20 normozoospermic patients. Graph reports the MDA intracellular concentration as a marker of oxidative damage in normozoospermic samples (normo), asthenozoospermic samples (astheno) and asthenozoospermic samples lasered and immediately evaluated (astheno + laser T0) or assessed after 30 min (astheno + laser T 30). Data are expressed as mean ± SD. Statistical analysis was performed using one-way ANOVA followed by Tukey’s multiple comparison test. ** and *** indicate a significant difference (p < 0.01 and 0.001, respectively) between normo and astheno, astheno + laser T0), or astheno + laser T 30. No significant differences have been observed between astheno versus astheno + laser T0 or astheno + laser T30.
The positive contribution of PBM on sperm parameters has been reported in several studies20,32,33,34. However, while PBM offers a translational potential to treat spermatozoa with poor motility, there is no common standard, making it difficult to directly compare the various data and draw a firm conclusion about the safety of PBM on semen samples in clinical practice. Our results showed that laser irradiation of human asthenozoospermic samples significantly improves progressive motility through increased mitochondrial energetic metabolism without harmful oxidative stress nor negative impact on sperm membrane integrity and chromatin condensation.
We used a diode laser light of 810 nm that we previously demonstrated to induce PBM on Paramecium primaurelia and mammalian mitochondrial activity without generating thermal damage13,60,61. It also did not negatively affect fertilization and early embryo development after irradiation of sea urchin eggs and spermatozoa58. Moreover, other Authors reported promising results at 810 nm in human normozoospermic semen samples41. After identifying 1 W and 2 W as the most influential powers in increasing progressive motility and decreasing the percentage of immotile sperm, we chose 1 W for the following experiments. Indeed, the therapy showed preliminary aspects of efficacy and safety based on our evidence in vitro on protozoa and in vivo on sea urchins and humans. Indeed, 810 nm 1 W for 60 s over an area of 1 cm2 increased the ATP production and the movement of the cilia in Paramecium primaurelia62,63; the mechanisms underlying the movement of the cilia in protozoa have homologies with the flagellar movement of spermatozoa64,65. It also improved fertilization rates in healthy sea urchin spermatozoa58, and allowed complete recovery in patients with Bell’s palsy who were unresponsive to drugs66. No adverse effects were reported after follow-up.
We focused exclusively on a population of asthenozoospermic patients to avoid possible confounding factors. Specifically, we considered that normozoospermic samples could not benefit from laser therapy or do so in a reduced way. On the other hand, the low sperm number in oligozoospermic samples may make it challenging to count enough sperm to appreciate the laser effect on motility. To the best of our knowledge, this is the first study that included only fresh abnormal sperm and enrolled the largest cohort of semen samples from asthenozoospermic patients.
Semen parameters of asthenozoospermic patients were shifted above a threshold of 30% progressive motility, representing the standard criteria for normozoospermic, while in parallel, the fraction of immotile sperm decreased. Our results are in accordance with previous publications that reported enhanced sperm motility in semen samples treated with an infrared range of PBM36,39,41.
The underlying pathways of PBM therapy are not well established and may vary among different sperm states (fresh versus frozen, normal versus abnormal). At present, at least four mechanisms are believed to be related to the response of sperm to PBM: (i) changes in mitochondrial function, (ii) generation and/or release of reactive oxygen species (ROS) from sperm cells; (iii) formation of nitric oxide, (iv) activation of opsins coupled to G-proteins34. In this study, we investigated the involvement of mitochondria since they serve as the primary energy source for sperm movement, and in asthenozoospermia, sperm motility is impaired due to mitochondrial malfunction or structural defects of the tail8. In addition, the release of nitric oxide and the effects on the opsins are mainly the result of an interaction in the visible range of 450 to 650 nm19,67. Our PBM protocol increased mitochondrial energy metabolism, providing biochemical evidence that mitochondrial activation is implicated in the increased sperm motility observed.
To broaden the knowledge of PBM treatment of human sperm, it is essential to analyze side effects. To date, it is challenging to draw a firm conclusion about the safety of PBM on semen samples in male subjects because there are some significant differences in the data available in the literature in terms of population characteristics, sample size, and type of methods used to conduct safety tests. In general, most of the human sperm DNA damage during manipulation is oxidatively induced68 because spermatozoa are poor in enzymes that metabolize ROS, like catalase and glutathione peroxidase68,69 and have limited DNA repair capability70. This may be why 810 nm PBM did not reduce oxidative stress damage in irradiated asthenozoospermic samples, as has been observed in other cell types71.
Oxidative stress causes lipid peroxidation of the plasma membrane, which is rich in polyunsaturated fatty acids, leading to the breakdown of membrane-dependent functions in these cells, including motility and sperm‐oocyte fusion70. Since we did not observe MDA accumulation, a marker of lipidic peroxidation, in irradiated asthenozoospermic samples, we argue that our PBM treatment does not increase oxidative stress in sperm. Noteworthy, to investigate this aspect without introducing potential biases into the system, we applied PBM only to native spermatozoa within the seminal plasma since manipulation (centrifugation, resuspension) of seminal fluids results in an artificially high level of ROS72,73. Moreover, seminal plasma is one of the most potent antioxidant fluids70.
Functionally, our laser therapy protocol did not adversely affect the integrity of the sperm membrane, preserving vitality and chromatin condensation, and did not introduce DNA fragmentation or worsen it when already present.
All these safety aspects are essential for applying PBM in clinical practice as an asthenozoospermia therapy. One of the putative applications would be a laser-induced improvement of sperm motility to increase pregnancy rates achieved by intrauterine insemination. The percentage of motile sperm that has the ability for forward progression is one of the most critical prognostic indicators of fertilization, which can increase the likelihood of pregnancy74. Bypassing time, sperm cells decrease their ability to move75. Boosting the availability of total motile sperm can improve the chance of pregnancy. This finding could be helpful in cases of moderate male infertility to encourage the use of intrauterine insemination as a cost-effective first-line treatment76. On the one hand, it is noteworthy that in our experiments, the effect of irradiation on sperm motility was kept high within 30 min after irradiation, unlike other studies where the effect decreased significantly after 10 min of phototherapy77. On the other hand, we have shown a significant increase in progressive sperm motility from 29 to 35%, but we cannot conclude that this increase predicts a better clinical outcome.
More benefits could be expected in selecting viable ejaculated or surgically retrieved testicular/epididymal spermatozoa in patients with immotile sperm before microinjection into the oocyte. Sperm motility is an essential selection criterion for embryologists during intracytoplasmic sperm injection (ICSI). A method of testing sperm viability is to induce sperm motility by increasing cyclic adenosine monophosphate (cAMP) levels by treating a semen sample with phosphodiesterase inhibitors, such as theophylline and pentoxifylline78. Although pentoxifylline is a popular in vitro sperm motility enhancer in many embryology laboratories, its application is not commonly approved in clinical practice because the consequences of pentoxifylline treatments in terms of potential damage to sperm DNA, oocytes, embryos, and newborns are contradictory79. In this context, stimulating sperm motility by PBM could advance sperm selection.
Another clinical application of PBM could be the treatment of frozen and thawed semen, especially in programs of fertility preservation of cancer patients before chemo- and radiotherapy. Despite its widespread use, the cryopreservation technique can cause damage to sperm through oxidative stress that induces lipid peroxidation, DNA fragmentation, and apoptosis80. In addition, sperm motility and fertilizing potential are reduced due to membrane, cytoskeletal, and acrosome damage81,82. The harmful side effects of the freezing and thawing process may be countered by PBM-preconditioning of fresh semen before the cryopreservation procedure, hopefully resulting in increased mitochondrial membrane potential and decreased levels of intracellular ROS and lipid peroxidation45,46.
Of course, some ethical concerns must be resolved before the use of PBM of sperm in assisted reproductive technologies (ART), whose ultimate goal is a healthy baby. Indeed, laser technology is now being applied in ART to reduce procedure times and increase the consistency of traditional techniques such as assisted hatching, embryo biopsy in the preimplantation genetic diagnosis program, and sperm immobilization83. It is also important to note that the energy used to enhance sperm motility in PBM is much lower than those used for, i.e., assisted hatching, since it is only used to stimulate the photosensitive enzyme cytochrome c oxidase and below the threshold for ionization. However, there is undoubtedly little published information about the potential adverse effects that laser application - even for PBM of sperm - could have on the developing human embryo, making further research necessary.
Overall, our work encompasses both advantages and limitations. First of all, we explored very deeply the specific mechanism behind the positive effect of PBM on sperm motility. In fact, the analysis of energy metabolism in irradiated and non-irradiated asthenozoospermic spermatozoa and the comparison with healthy spermatozoa clearly showed that the macroscopic effect on cell motility is a consequence of the impact of our light therapy on mitochondrial oxidative phosphorylation. However, the glycolytic pathway, also known to play an energetic role in sperm motility, does not appear to be activated by light, as well as opsins and nitric oxide are predominantly stimulated in the visible range. Second, the strength of our work lies in our approach, which represents the first standardisable protocol easily adaptable to the clinical environment, shifting the focus from preliminary data and evidence towards a coherent and consistent demonstration of the photobiomodulation phenomenon in the context of male infertility. In fact, the short exposure time prevents samples from being exposed to sub-optimal conditions for too long, and the immediate effect, which lasts consistently up to 30 min (after 60 min, the effect returns to the level of untreated samples), ensures consistency of the procedure. Additionally, using an innovative FT-HP, which we have previously characterized, to deliver photon energy allowed irradiation to be independent of distance from the target and uniform across the entire 1 cm2 surface area. These properties make the approach repeatable, operator-independent and more effective than standard delivery systems used in photobiomodulatory therapy48,49.
We are aware that there are some limitations. First, in this study, we checked the number of spermatozoa and sperm motility by microscopic investigation. However, the method followed the practical guidelines in the WHO manual. We know semen examination is complicated and procedurally challenging to standardize, mainly because it is based on operator-dependent procedures. Anyway, the accuracy of the data shown in this study was ensured because in our laboratory we monitor the fundamental parameters of sperm count, morphology, and motility through internal and external quality control procedures. Our operators are qualified, and performance is periodically monitored to detect systematic and random differences between individuals performing analyses. Moreover, our laboratory, as the Regional referral center for semen diagnostic analysis, adheres to an external quality verification program to detect systematic variations and evaluate accuracy through comparisons between different laboratories. In addition, to limit bias, semen parameters were assessed blindly and through commercial disposables and kits for clinical use to provide repeatable and consistent results.
A second limitation is that we did not check the straightness of sperm movement and the flagellar waveform, which helps evaluate sperm hyperactivation84. However, the complex parameters of the flagellar movement of hyperactivated spermatozoa are challenging to identify reliably and unambiguously through manual analysis. Motility stereotypes can be assessed by computer-aided sperm analysis (CASA). However, the comparability of measurements among different instruments is not yet known, and their biological value is not fully understood51. Lastly, we did not evaluate the effectiveness of our PBM protocol in improving fertilization potential and the impact on embryo quality. This was due to the Italian law prohibiting the insemination of human oocytes outside the standard procedures recognized as non-experimental. However, in the sea urchin, a well-known model for studying the molecular mechanisms underlying fertilization and human embryonic development85, we have obtained an encouraging increase in sperm fertilization rates, without the occurrence of embryonic and larval anomalies58.
Laser irradiation at 810 nm through the FT-HP of human sperm significantly improves progressive motility. The effect is due to increased metabolic energy (ATP) resulting from photons interacting with the OxPhos system instead of the glycolytic pathway represented by lactate dehydrogenase (LDH). What makes these data even more interesting is that the treatment did not cause harmful oxidative stress or negative effects on membrane integrity and chromatin condensation.
The data that support the findings of this study are available from the corresponding author upon reasonable request.
Agarwal, A., Mulgund, A., Hamada, A. & Chyatte, M. <ArticleTitle Language=“En”>R. A unique view on male infertility around the globe. Reproductive Biology Endocrinol. 13, 1–9 (2015).
Levine, H. et al. Temporal trends in sperm count: a systematic review and meta-regression analysis of samples collected globally in the 20th and 21st centuries. Hum. Reprod. Update. 29, 157–176 (2023).
Amaral, A., Lourenço, B., Marques, M. & Ramalho-Santos, J. Mitochondria functionality and sperm quality. Reproduction. 146, R163–R174 (2013).
Article CAS PubMed Google Scholar
Boguenet, M., Bouet, P. E., Spiers, A. & Reynier, P. May-Panloup, P. Mitochondria: their role in spermatozoa and in male infertility. Hum. Reprod. Update. 27, 697–719 (2021).
Article CAS PubMed Google Scholar
Gu, N. H., Zhao, W. L., Wang, G. S. & Sun, F. Comparative analysis of mammalian sperm ultrastructure reveals relationships between sperm morphology, mitochondrial functions and motility. Reprod. Biol. Endocrinol. 17, (2019).
Du Plessis, S. S., Agarwal, A., Mohanty, G. & Van Der Linde, M. Oxidative phosphorylation versus glycolysis: what fuel do spermatozoa use? Asian J. Androl. 17, 230–235 (2015).
Article CAS PubMed Google Scholar
Mukai, C. & Travis, A. J. What Sperm Can Teach us About Energy Production. Reprod. Domest. Anim. 47, 164 (2012).
Article PubMed PubMed Central Google Scholar
Piomboni, P., Focarelli, R., Stendardi, A., Ferramosca, A. & Zara, V. The role of mitochondria in energy production for human sperm motility. Int. J. Androl. 35, 109–124 (2012).
Article CAS PubMed Google Scholar
Karu, T. I., Letokhov, V. S. & Lobko, V. V. Biostimulation of HeLa cells by low-intensity visible light - IV.-Dichromatic irradiation. Il Nuovo Cimento D. 5, 483–496 (1985).
Karu, T. I. Special issue papers. photobiological fundamentals of low-power laser therapy. IEEE J. Quantum Electron. 23, 1703–1717 (1987).
Passarella, S., Ostuni, A., Atlante, A. & Quagliariello, E. Increase in the adp/atp exchange in rat liver mitochondria irradiated in vitro by helium-neon laser. Biochem. Biophys. Res. Commun. 156, 978–986 (1988).
Article CAS PubMed Google Scholar
Ravera, S. et al. 1064 nm Nd:YAG laser light affects transmembrane mitochondria respiratory chain complexes. J. Biophotonics 12, (2019).
Amaroli, A. et al. An 808-nm Diode Laser with a Flat-Top Handpiece Positively Photobiomodulates Mitochondria Activities. Photomed. Laser Surg. 34, (2016).
Amaroli, A., Clemente Vargas, M. R., Pasquale, C., Raffetto, M. & Ravera, S. Photobiomodulation on isolated mitochondria at 810 nm: first results on the efficiency of the energy conversion process. Sci. Rep. 14, (2024).
Amaroli, A. et al. Electromagnetic Dosimetry for Isolated Mitochondria Exposed to Near-Infrared Continuous-Wave Illumination in Photobiomodulation Experiments. Bioelectromagnetics. 42, 384–397 (2021).
Article CAS PubMed PubMed Central Google Scholar
Amaroli, A. et al. Photobiomodulation and Oxidative Stress: 980 nm Diode Laser Light Regulates Mitochondrial Activity and Reactive Oxygen Species Production. Oxid Med Cell Longev (2021).
Cassano, P. et al. Transcranial Photobiomodulation for the Treatment of Major Depressive Disorder. The ELATED-2 Pilot Trial. Photomed. Laser Surg. 36, 634–646 (2018).
Article PubMed PubMed Central Google Scholar
Amaroli, A. et al. Steering the multipotent mesenchymal cells towards an anti-inflammatory and osteogenic bias via photobiomodulation therapy: How to kill two birds with one stone. J. Tissue Eng. 13, (2022).
Colombo, E. et al. Experimental and Clinical Applications of Red and Near-Infrared Photobiomodulation on Endothelial Dysfunction: A Review. Biomedicines 9, (2021).
Eghbaldoost, A., Mashhadsari, S. P. S., Ghadirzadeh, E., Ghoreifi, A. & Allameh, F. Therapeutic Effects of Low-Level Laser on Male Infertility: A Systematic Review. J. Lasers Med. Sci. 14, (2023).
Ravera, S. et al. Mitochondrial bioenergetic, photobiomodulation and trigeminal branches nerve damage, what’s the connection? A review. Int. J. Mol. Sci. 22, 4347 (2021).
Article CAS PubMed PubMed Central Google Scholar
Hasani, A. et al. Photobiomodulation restores spermatogenesis in the transient scrotal hyperthermia-induced mice. Life Sci. 254, 117767 (2020).
Article CAS PubMed Google Scholar
Rezaei, F. et al. Photobiomodulation Therapy Improves Spermatogenesis in Busulfan-Induced Infertile Mouse. Reproductive Sci. 28, 2789–2798 (2021).
Ziaeipour, S. et al. Photobiomodulation therapy reverses spermatogenesis arrest in hyperthermia-induced azoospermia mouse model. Lasers Med. Sci. 38, 1–9 (2023).
Corral-Baques , MI , Rigau , T. , Rivera , M. , Rodriguez , JE & Rigau , J. Effect of 655-nm diode laser on dog sperm motility . Lasers With. Sci. 20, 28–34 (2005).
Corral-Baqués, M. I., Rivera, M. M., Rigau, T., Rodríguez-Gil, J. E. & Rigau, J. The effect of low-level laser irradiation on dog spermatozoa motility is dependent on laser output power. Lasers Med. Sci. 24, 703–713 (2009).
Fernandes, G. H. C. et al. The Effect of Low-Level Laser Irradiation on Sperm Motility, and Integrity of the Plasma Membrane and Acrosome in Cryopreserved Bovine Sperm. PLoS One. 10, e0121487 (2015).
Article PubMed PubMed Central Google Scholar
Iaffaldano, N. et al. Helium-neon laser irradiation of cryopreserved ram sperm enhances cytochrome c oxidase activity and ATP levels improving semen quality. Theriogenology. 86, 778–784 (2016).
Article CAS PubMed Google Scholar
Siqueira, A. F. P. et al. Effects of photobiomodulation therapy (PBMT) on bovine sperm function. Lasers Med. Sci. 31, 1245–1250 (2016).
Yeste, M., Castillo-Martín, M., Bonet, S. & Rodríguez-Gil, J. E. Impact of light irradiation on preservation and function of mammalian spermatozoa. Anim. Reprod. Sci. 194, 19–32 (2018).
Article CAS PubMed Google Scholar
Zan-Bar, T. et al. Influence of Visible Light and Ultraviolet Irradiation on Motility and Fertility of Mammalian and Fish Sperm. https://home.liebertpub.com/pho23, 549–555 (2005).
Zupin, L. et al. Photobiomodulation therapy for male infertility. Lasers Med. Sci. 35, 1671–1680 (2020).
Poorhassan, M. et al. Preclinical and Clinical Applications of Photobiomodulation Therapy in Sperm Motility: A Narrative Review. J. Lasers Med. Sci. 13, (2022).
Xue, Y., Xiong, Y., Cheng, X. & Li, K. Applications of laser technology in the manipulation of human spermatozoa. Reproductive Biology Endocrinol. 21, 1–17 (2023).
Panahi, Y., Alishiri, G. H., Parvin, S. & Sahebkar, A. Mitigation of Systemic Oxidative Stress by Curcuminoids in Osteoarthritis: Results of a Randomized Controlled Trial. J. Diet. Suppl. 13, 209–220 (2016).
Article CAS PubMed Google Scholar
Lenzi, A. et al. Laser Radiation and Motility Patterns of Human Sperm. Arch. Androl. 23, 229–234 (1989).
Article CAS PubMed Google Scholar
Singer, R. et al. Low energy narrow band non-coherent infrared illumination of human semen and isolated sperm. Andrologia 23, 181–184 .
Sato, H., Landthaler, M., Haina, D. & Schill, W. B. The effects of laser light on sperm motility and velocity in vitro. Andrologia. 16, 23–25 (1984).
Firestone, R. S. et al. The effects of low-level laser light exposure on sperm motion characteristics and DNA damage. J. Androl. 33, 469–473 (2012).
Article CAS PubMed Google Scholar
Preece, D. et al. Red light improves spermatozoa motility and does not induce oxidative DNA damage. Sci. Rep. 7, (2017).
Safian, F. et al. Photobiomodulation with 810 nm Wavelengths Improves Human Sperms’ Motility and Viability In Vitro. Photobiomodul Photomed. Laser Surg. 38, 222–231 (2020).
Espey, B. T. et al. Effects of Pulsed-Wave Photobiomodulation Therapy on Human Spermatozoa. Lasers Surg. Med. 54, 540–553 (2022).
Salman Yazdi, R. et al. Effect of 830-nm diode laser irradiation on human sperm motility. Lasers Med. Sci. 29, 97–104 (2014).
Ahmed, R., Hamdy, O., Elattar, S. & Soliman, A. A. Improving human sperm motility via red and near-infrared laser irradiation: in-vitro study. Photochem. Photobiol Sci. 23, 377–385 (2024).
Article CAS PubMed Google Scholar
Safian, F. et al. Comparative Effect of Photobiomodulation on Human Semen Samples Pre- and Post-Cryopreservation. Reprod. Sci. 29, 1463–1470 (2022).
Article CAS PubMed Google Scholar
Safian, F. et al. Photobiomodulation preconditioned human semen protects sperm cells against detrimental effects of cryopreservation. Cryobiology. 98, 239–244 (2021).
Article CAS PubMed Google Scholar
Highland, H., Rajput, N., Sharma, R. & George, L. B. Differential sensitivity of the human sperm cell to near infrared radiation. J. Photochem. Photobiol B. 183, 119–126 (2018).
Article CAS PubMed Google Scholar
Amaroli, A. et al. Improving Consistency of Photobiomodulation Therapy: A Novel Flat-Top Beam Hand-Piece versus Standard Gaussian Probes on Mitochondrial Activity. Int. J. Mol. Sci. 22, (2021).
Hanna, R. et al. A comparative study between the effectiveness of 980 nm photobiomodulation delivered by hand-piece with Gaussian vs. Flat-top profiles on osteoblasts maturation. Front. Endocrinol. (Lausanne) 10, (2019).
Shrestha, B. & Dunn, L. The Declaration of Helsinki on Medical Research involving Human Subjects: A Review of Seventh Revision. J. Nepal. Health Res. Counc. 17, 548–552 (2020).
WHO laboratory manual for the examination and processing of human semen. https://iris.who.int/handle/10665/343208
Bertola, N. et al. Effects of Deacetylase Inhibition on the Activation of the Antioxidant Response and Aerobic Metabolism in Cellular Models of Fanconi Anemia. Antioxid. (Basel) 12, (2023).
Ravera, S. et al. Identification of biochemical and molecular markers of early aging in childhood cancer survivors. Cancers (Basel) 13, (2021).
Ravera, S. et al. Evaluation of energy metabolism and calcium homeostasis in cells affected by Shwachman-Diamond syndrome. Sci. Rep. 6, (2016).
Ravera, S. et al. Assessing the Effects of Curcumin and 450 nm Photodynamic Therapy on Oxidative Metabolism and Cell Cycle in Head and Neck Squamous Cell Carcinoma: An In Vitro Study. Cancers 2024. 16, 1642 (2024).
Bertola, N., Degan, P., Cappelli, E. & Ravera, S. Mutated FANCA Gene Role in the Modulation of Energy Metabolism and Mitochondrial Dynamics in Head and Neck Squamous Cell Carcinoma. Cells 11, (2022).
Bernard Rosner. Fundamentals of Biostatistics, 8th Edition - Bernard Rosner - Cengage Learning - 978-1305268920. 927. (2015).
Amaroli, A. et al. The Effect of Photobiomodulation on the Sea Urchin Paracentrotus lividus (Echinodermata) Using Higher-Fluence on Fertilization, Embryogenesis, and Larval Development: An in Vitro Study. Photomed. Laser Surg. 35, (2017).
Amaroli, A., Clemente Vargas, M. R., Pasquale, C., Raffetto, M. & Ravera, S. Photobiomodulation on isolated mitochondria at 810 nm: first results on the efficiency of the energy conversion process. Scientific Reports 2024 14:1 14, 1–11 (2024).
Amaroli, A. et al. 808-nm laser therapy with a flat-top handpiece photobiomodulates mitochondria activities of Paramecium primaurelia (Protozoa). Lasers Med. Sci. 31, 741–747 (2016).
Andrea, A. et al. The protozoan, Paramecium primaurelia, as a non-sentient model to test laser light irradiation: The effects of an 808nm infrared laser diode on cellular respiration. Altern. Lab. Anim. 43, 155–162 (2015).
Amaroli, A. et al. Photobiomodulation by Infrared Diode Laser: Effects on Intracellular Calcium Concentration and Nitric Oxide Production of Paramecium. Photochem. Photobiol 92, (2016).
Amaroli, A. et al. Effect of 808 nm Diode Laser on Swimming Behavior, Food Vacuole Formation and Endogenous ATP Production of Paramecium primaurelia (Protozoa). Photochem. Photobiol 91, 1150–1155 .
Hamel, A., Fisch, C., Combettes, L., Dupuis-Williams, P. & Baroud, C. N. Transitions between three swimming gaits in Paramecium escape. Proc. Natl. Acad. Sci. U S A. 108, 7290–7295 (2011).
Article ADS CAS PubMed PubMed Central Google Scholar
Linck, R. W. Cilia and Flagella. in eLS 1–17 (John Wiley & Sons, Ltd, Chichester, UK https://doi.org/10.1002/9780470015902.a0001258.pub3 (2015).
et al. Recovery from Idiopathic Facial Paralysis (Bell’s Palsy) Using Photobiomodulation in Patients Non-Responsive to Standard Treatment: A Case Series Study. Photonics 2021, Vol. 8, Page: 341 8, 341 (2021).
Suh, S. & Choi, E. H. Atanaskova Mesinkovska, N. The expression of opsins in the human skin and its implications for photobiomodulation: A Systematic Review. Photodermatol Photoimmunol Photomed. 36, 329 (2020).
Article PubMed PubMed Central Google Scholar
Aitken, R. J., Gibb, Z., Baker, M. A., Drevet, J. & Gharagozloo, P. Causes and consequences of oxidative stress in spermatozoa. Reprod. Fertil. Dev. 28, 1–10 (2016).
Article CAS PubMed Google Scholar
Agarwal, A., Saleh, R. A. & Bedaiwy, M. A. Role of reactive oxygen species in the pathophysiology of human reproduction. Fertil. Steril. 79, 829–843 (2003).
Aitken, R. J. Reactive oxygen species as mediators of sperm capacitation and pathological damage. Mol. Reprod. Dev. 84, 1039–1052 (2017).
Article CAS PubMed Google Scholar
Rupel, K. et al. Photobiomodulation at Multiple Wavelengths Differentially Modulates Oxidative Stress In Vitro and In Vivo. Oxid Med Cell Longev 6510159 (2018).
Moein, M. R., Vahidi, S., Ghasemzadeh, J. & Tabibnejad, N. Comparison of reactive oxygen species in neat and washed semen of infertile men. Iran. J. Reprod. Med. 12, 301 (2014).
PubMed PubMed Central Google Scholar
Castleton, P. E., Deluao, J. C., Sharkey, D. J. & McPherson, N. O. Measuring Reactive Oxygen Species in Semen for Male Preconception Care: A Scientist Perspective. Antioxid. (Basel) 11, (2022).
Miller, D. C. et al. Processed total motile sperm count correlates with pregnancy outcome after intrauterine insemination. Urology. 60, 497–501 (2002).
Valsa, J., Skandhan, K. P., Sumangala, B. & Jaya, V. Time bound changes (in 24 h) in human sperm motility and level of calcium and magnesium in seminal plasma. Alexandria J. Med. 52, 235–241 (2016).
Ombelet, W. et al. Semen quality and intrauterine insemination. Reprod. Biomed. Online. 7, 485–492 (2003).
Salama, N. & El-Sawy, M. Light-emitting diode exposure enhances sperm motility in men with and without asthenospermia: preliminary results. Arch. Ital. Urol. Androl. 87, 14–19 (2015).
Article CAS PubMed Google Scholar
Gala, B. et al. The Potential of Theophylline and Pentoxifylline in Sperm Optimization and Its Intracytoplasmic Sperm Injection Outcomes. Cureus 15, (2023).
Mahaldashtian, M. et al. Does in vitro application of pentoxifylline have beneficial effects in assisted male reproduction? Andrologia 53, (2021).
Gualtieri, R. et al. Mitochondrial Dysfunction and Oxidative Stress Caused by Cryopreservation in Reproductive Cells. Antioxid. (Basel). 10, 1–23 (2021).
Wang, S. et al. Proteomic characteristics of human sperm cryopreservation. Proteomics. 14, 298–310 (2014).
Article CAS PubMed Google Scholar
Bogle, O. A. et al. Identification of protein changes in human spermatozoa throughout the cryopreservation process. Andrology. 5, 10–22 (2017).
Article CAS PubMed Google Scholar
Davidson, L. M., Liu, Y., Griffiths, T., Jones, C. & Coward, K. Laser technology in the ART laboratory: a narrative review. Reprod. Biomed. Online. 38, 725–739 (2019).
Mortimer, S. T. A critical review of the physiological importance and analysis of sperm movement in mammals. Hum. Reprod. Update. 3, 403–439 (1997).
Article CAS PubMed Google Scholar
Bernardo, M., Di, Carlo, M., Di Di, Bernardo, M. & Carlo, M. Di. The Sea Urchin Embryo: A Model for Studying Molecular Mechanisms Involved in Human Diseases and for Testing Bioactive Compounds. Sea Urchin - Environ. Aquaculture Biomed. https://doi.org/10.5772/INTECHOPEN.70301 (2017).
We thank Eng. Cucinotta of Garda Laser for his key role in providing the ENEA diode laser (GaAlAs) and for his commitment to the advancement of scientific research.
This work was partially supported by the Fondo per la Ricerca di Ateneo (FRA) University of Genova, code 100022-2023-AA-FRA_001 to A.A.
Andrea Amaroli and Paola Scaruffi contributed equally to this work.
SS Physiopathology of Human Reproduction, IRCCS Policlinico San Martino Hospital, Genoa, Italy
Sara Stigliani, Elena Maccarini, Camilla Rizzo, Claudia Massarotti, Paola Anserini & Paola Scaruffi
Experimental Medicine Department, University of Genova, Genova, Italy
IRCCS Policlinico San Martino Hospital, Genoa, Italy
Department of Neuroscience, Rehabilitation, Ophthalmology, Genetics and Maternal-Child Health (DiNOGMI), University of Genova, Genova, Italy
BIO-Photonics Overarching Research laboratory, Department of Earth, Environmental and Life Sciences (DISTAV), University of Genova, Genova, Italy
Matteo Bozzo & Andrea Amaroli
You can also search for this author in PubMed Google Scholar
You can also search for this author in PubMed Google Scholar
You can also search for this author in PubMed Google Scholar
You can also search for this author in PubMed Google Scholar
You can also search for this author in PubMed Google Scholar
You can also search for this author in PubMed Google Scholar
You can also search for this author in PubMed Google Scholar
You can also search for this author in PubMed Google Scholar
You can also search for this author in PubMed Google Scholar
Conceptualization, Data curation, Formal analysis, S.S; S.R.; A.A.; P.S. Methodology, S.S; S.R.; E.M.; C.R.; A.A.; P.S. Funding acquisition, A.A.; P.A. Visualization, S.R.; M.B.; A.A.; P.S.; Writing-original draft, S.R.; A.A.; P.S; Writing-review and editing, S.S., A.A.; P.S.; Sofware and Resources, C.M.; M.B.
Correspondence to Silvia Ravera or Andrea Amaroli.
The authors declare no competing interests.
Ethics Committee of Liguria Region (219/2024 - DB ID 13852).
Written informed consent was obtained from all donors, and participation was voluntary.
Springer Nature remains neutral with regard to jurisdictional claims in published maps and institutional affiliations.
Open Access This article is licensed under a Creative Commons Attribution-NonCommercial-NoDerivatives 4.0 International License, which permits any non-commercial use, sharing, distribution and reproduction in any medium or format, as long as you give appropriate credit to the original author(s) and the source, provide a link to the Creative Commons licence, and indicate if you modified the licensed material. You do not have permission under this licence to share adapted material derived from this article or parts of it. The images or other third party material in this article are included in the article’s Creative Commons licence, unless indicated otherwise in a credit line to the material. If material is not included in the article’s Creative Commons licence and your intended use is not permitted by statutory regulation or exceeds the permitted use, you will need to obtain permission directly from the copyright holder. To view a copy of this licence, visit http://creativecommons.org/licenses/by-nc-nd/4.0/.
Stigliani , S. , Ravera , S. , Maccarini , E. et al. The power of 810 nm near-infrared photobiomodulation therapy for human asthenozoospermia. Sci Rep 14, 26819 (2024). https://doi.org/10.1038/s41598-024-77823-7
DOI: https://doi.org/10.1038/s41598-024-77823-7
Anyone you share the following link with will be able to read this content:
Sorry, a shareable link is not currently available for this article.
Provided by the Springer Nature SharedIt content-sharing initiative
Scientific Reports (Sci Rep) ISSN 2045-2322 (online)
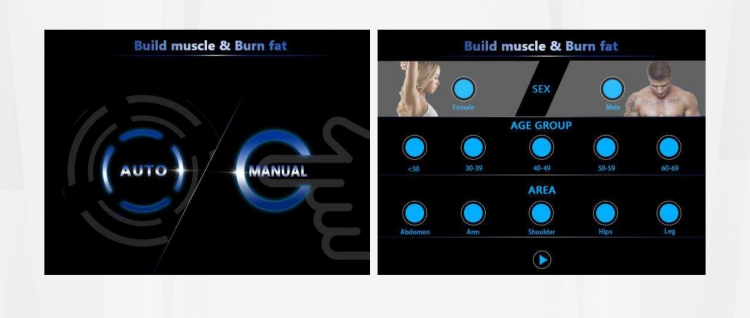
Best Laser Hair Removal Sign up for the Nature Briefing newsletter — what matters in science, free to your inbox daily.