Dear user, ET HealthWorld privacy and cookie policy has been updated to align with the new data regulations in European Union. Please review and accept these changes below to continue using the website. You can see our privacy policy & our cookie policy. We use cookies to ensure the best experience for you on our website. If you choose to ignore this message, we'll assume that you are happy to receive all cookies on ET HealthWorld.
Novartis: will not move forward with UK anti-cholesterol drug trial1 hr ago N-Boc-L-Pyroglutamic Acid Ethyl Ester

Roche teams up with Lilly to validate Alzheimer's blood test2 hrs ago
The unsung hero in faster holistic recovery
Technological Disruption in Indian Health & Pharma
Do you think you are up for the challenge?
Holistic Solutions For A Healthier India
New Delhi, Apr 25 (PTI) Gufic Biosciences on Monday said it has received an approval from the Central Drugs Standard Control Organisation (CDSCO) to manufacture and market a drug used to treat invasive Aspergillosis and Mucormycosis.
Join the community of 2M+ industry professionals
The Union Health Ministry stated in Parliament today that there is a 71 per cent increase in medical colleges from 387 before 2014 to 660 as of now. Indicated that 101,043 seats are currently available, up from 51,348 in 2014, of which 52,778 are in government medical institutions and the remaining 48,265 are in private medical colleges.
The Union Health Ministry stated in Parliament today that there is a 71 per cent increase in medical colleges from 387 before 2014 to 660 as of now. Indicated that 101,043 seats are currently available, up from 51,348 in 2014, of which 52,778 are in government medical institutions and the remaining 48,265 are in private medical colleges.
We have various options to advertise with us including Events, Advertorials, Banners, Mailers, Webinars etc.
Please contact us to know more details.
Get ETHealthworld 's top stories every morning in your email inbox.
Join the community of 2M+ industry professionals
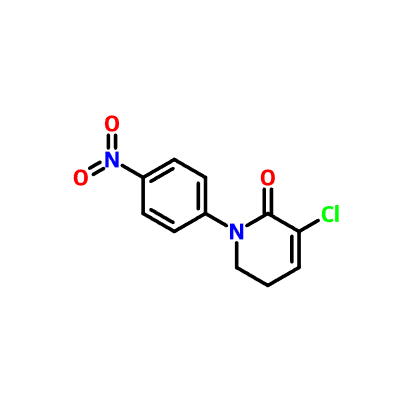
Betadex Sulfobutyl Ether Soidum Follow @ETHealthworld for the latest news, insider access to events and more.