Thank you for visiting nature.com. You are using a browser version with limited support for CSS. To obtain the best experience, we recommend you use a more up to date browser (or turn off compatibility mode in Internet Explorer). In the meantime, to ensure continued support, we are displaying the site without styles and JavaScript.
Scientific Reports volume 13, Article number: 18009 (2023 ) Cite this article Ink Cartridge
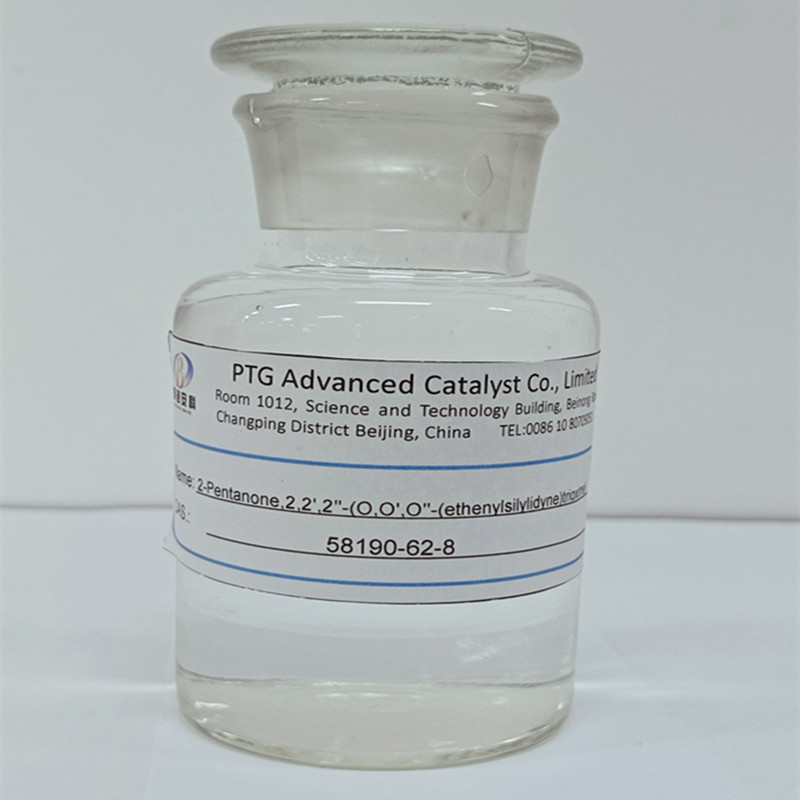
Deep eutectic solvents have countless advantages over normal solvents, and in addition to complying with the principles of green chemistry, depending on their nature, they can also act as catalysts. The use of deep eutectic solvents as acid catalysts has several advantages such as non-toxicity, a catalytic effect similar to or higher than the acid itself, and the possibility of recovery and reuse without significant loss of activity. In this project, A novel deep eutectic solvent (MTPPBr–PCAT–DES) was prepared from a one-to-one mole ratio of methyltriphenyl-phosphonium bromide (MTPPBr) and 3,4-dihydroxybenzoic acid (PCAT = protocatechuic acid) and characterized by various techniques such as FT-IR, TGA/DTA, densitometer, eutectic point, 1H NMR, 13C NMR and 31P NMR. Then, it was used as a novel and capable catalyst for the synthesis of pyranopyrimidines from the multicomponent condensation reaction of barbituric acid, 4-hydroxycoumarin, and aromatic aldehydes in mild conditions, short reaction times, and high yields.
Hadis Goudarzi, Davood Habibi & Arezo Monem
Mehri Moeini Korbekandi, Iraj Mohammadpoor-Baltork, ... Behrouz Notash
Jamal Rahimi, Maryam Niksefat, … Ali Maleki
The attention of scientists and industrial interests has been attracted by the development of simple, efficient, green, and low-cost methods for the synthesis of organic compounds in this century. Although convention strategies are primarily focused on high-throughput methods in the shortest time, modern methods are eager to improve reusability, avoid waste generated, and minimize toxicity. To minimize the amount of toxic byproducts, hazardous reagents should be replaced with safer sources and greener and more environmentally friendly methods1. The concept of "green chemistry" refers to the means of harnessing natural resources to improve reaction efficiency, including the development and implementation of new chemical processes and transformations that are more efficient, safer, and more environmentally friendly2,3. Solvents have a strategic position thanks to the green chemistry framework. The solvent of a green environment should have sundry criteria such as availability, non-toxicity, recyclability, thermal stability, non-flammability, renewable, low vapor pressure, frugality, and bio-degradability4,5.
Deep eutectic solvents (DES) were first reported by the Abbott research group6. These compounds are a mixture of hydrogen bond acceptor groups (HBA) such as ammonium or phosphonium-based salts and hydrogen bond donor compounds (HBD) such as alcohols, amines and acids, which are synthesized under mild conditions6,7. DESs are an alternative class of ionic liquids (ILs) that have attracted the attention of many scientists in recent years8,9,10. The limitations of ILs are their toxicity, poor biodegradability, and high production cost, which DES have replaced as a new type of green solvent, and they do not have these limitations and are economically viable11,12. In addition, DES is easy to prepare and has many advantages such as high purity, low synthesis cost, high thermal stability, non-toxicity, reusability and bio-degradability. Moreover, they can be used as catalytically active species in many reactions13.
Therefore, the application and efficiency of DES as a green and environmentally friendly catalyst and or solvent in the synthesis of many compounds is of great importance and cannot be denied14,15,16,17. To date, various types of DES have been synthesized that offer significant advantages in organic synthesis.1
A multicomponent reaction (MCR) is a reaction in which three or more reagents are added simultaneously to a reaction flask and mixed in a one-pot process. These reactions have several advantages over linear synthesis protocols, including Fewer steps, and no need to isolate reaction intermediates (resulting in fewer purification steps), Since most of the carbon atoms are present in the final product, MCR can be considered as a very atom-economic process18,19,20.
N-heterocycles are organic compounds that contain at least one nitrogen atom in the ring, which often show unique properties and reactivities. These compounds have various applications in different fields such as pharmaceuticals, agricultural chemicals, and material science. In addition, they mainly have antibacterial, anti-tumor, anti-Alzheimer, anti-fungal, anti-inflammatory, anti-depressant, etc. properties. The appropriate methods for preparation of new heterocycles that can be used in various fields are the use of multi-component reactions, which increase the efficiency of these products and create appropriate economic benefits for the preparation of these compounds21,22,23,24,25,26,27,28,29,30,31. Among of N-heterocycles, pyranopyrimidine moiety widely exists in the structure of various natural products and has been shown to have various pharmacological activities such as antibronchitis32, antimicrobial33, anti-tumor34,35, antihyper-tensive, anticoagulant, antitumor, cardiac, liver protection, and anti-bronchitis activities36,37,38,39,40,41,42, and several methods reported for their syntheses43,44,45,46,47,48,49. Also, chromenes have many important biological properties such as anti-anaphylactic, anti-cancer, anti-coagulant, spasmolytic, diuretic, etc., and have received a lot of attention50,51,52,53,54. Therefore, the chromenopyrano[2,3-d]-pyrimidine system seems to be interesting because it has chromene and a pyranopyrimidine ring, both of which are promising for biological responses55. The synthesis of chromeno-pyranopyrimidines was first reported in 2014 by tetra-n-butylammonium tetrafluoroborate (TBAF) in CH3CN56.
In this report, the aim is to design and synthesize MTPPBr–PCAT–DES as a novel DES for the synthesis of pyranopyrimidines that have potential biological properties. Compared with other DESs, the synthesized DES has advantages such as an easy synthesis process, low melting point, high purity, non-toxicity, biodegradability, and lower price. Since deep eutectic solvents have found many applications, a novel Deep Eutectic Solvent (MTPPBr–PCAT–DES) was prepared from a one-to-one mole ratio of MTPPBr and PCAT (Scheme 1) and characterized by various techniques such as FT-IR, TGA/DTA, densitometer, eutectic point, and 1H NMR.
Synthesis of MTPPBr–PCAT–DES as a novel DES.
Then, it was used as a capable and novel catalyst for the synthesis of seven new 2(a-f) and five 2(g-l) known chromenopyranopyrimidines from the reaction of barbituric acid, 4-hydroxy- coumarin, and aromatic aldehydes in solvent-free conditions at 80 °C (Scheme 2).
Synthesis of 2(a-l) by MTPPBr–PCAT–DES.
The key advantages of this new protocol are the use of inexpensive DES as a green solvent and catalyst, a one-step MCR reaction, good to excellent yields, short reaction times, mild reaction conditions, and reusability, which have several aspects of green and sustainable chemistry.
All reagents were purchased from the chemical companies Merck or Sigma Aldrich and used as received. Thin layer chromatography (TLC) was performed on silica gel 60 F-254 [Merck, Germany]. NMR was recorded on a Bruker DRX-250 using DMSO-d6 as solvent. FT-IR (KBr) spectra were recorded using an Alpha Perkin Elmer spectrophotometer. Melting points were determined using a Stuart melting point meter. Thermo-Gravimetric-Differential Thermal Analysis (TGA–DTA) was performed using the SDT Q600 V20.9 Build 20 device. The density of the DES catalyst was determined using AND-HR200.
The mixture (molar ratio 1:1) of MTPPBr (1 mmol) and PCAT (1 mmol) was stirred at 90 °C in solvent-free conditions until a homogeneous liquid was obtained. When it cools slowly at room temperature, it turns into a transparent solid which dissolves well in water or ethanol.
MTPPBr–PCAT–DES (1.5 mmol), barbituric acid (1 mmol, 128 mg), 4-hydroxycoumarin (1 mmol, 174 mg), and aldehyde (1.0 mmol) were mixed in the presence of MTPPBr–PCAT–DES (1 mmol, 0.511 g) as a novel DES catalyst and stirred at 80 °C in solvent‐free condition for an appropriate time. After completion of the reaction (TLC), the resulting mixture was washed with ethanol and filtered to separate the catalyst from other materials (the reaction mixture is insoluble in ethanol and the catalyst is soluble). Upon completion of the reaction, a solid mass precipitated out and was filtered off, followed by the purification of the crude product just by washing it with cold aqueous ethanol.
The structure of each purified compound was confirmed with a comparison of their FT-IR, 1 HNMR, 13C NMR, and Mass spectra with authentic samples as well as their melting points.
Deep eutectic solvents are an emerging class of ionic liquids that have expanded in recent years as environmentally friendly compounds that play both the role of a solvent and a catalyst. The catalytic performance of these compounds is based on the creation of hydrogen bonds, which increase their selectivity. It should be noted that the synthesis method of deep eutectic solvents is very easy and they are synthesized by mixing and heating without the need for purification and separation. Therefore, the mixture of MTPPBr and PCAT (molar ratio 1:1) was heated at 90 °C until a homogeneous liquid (DES) was obtained.
The novel DES was characterized by FT-IR, TGA–DTA, densitometer, and eutectic points.
Figure 1 shows the FT-IR spectra of MTPPBr (a), PCAT (b), the fresh MTPPBr–PCAT–DES (DES catalyst) (c), and the recovered MTPPBr–PCAT–DES (DES catalyst) (d).
The FT-IR spectra of (a), (b), (c), and (d).
In spectrum (a), the peaks at about 2900–3100 cm−1 belong to the aromatic and aliphatic hydrogens, and the peaks at about 750 and 1480 cm−1 are related to the C-P bonds, respectively. In spectrum (b), the peaks at 3226 and 1675 cm−1 are related to the O–H and C=O of the –COOH group, respectively. The (c) spectrum shows the similar peaks shown in both (a) and (b) spectra confirming the structure of the DES catalyst. The peaks related to the O–H and C=O groups appeared with slight shifts at 3183 and 1697 cm−1, indicating the successful DES synthesis.
There is a nice similarity between the FT-IR spectra of (c), and (a) and (b) with slight shifts in the related peaks. As we know, when the hydrogen bond forms, the bond vibration requirs less energy, the vibration is easier and is transferred to a lower frequency (more wavelength) as can be seen in the FT-IR spectrum of (c) compared to the FT-IR spectra of (a) and (b).
In addition to other methods, the 1H NMR, 13C NMR, and 31P NMR techniques were also used for further confirmation.
Figure 2 shows the 1H NMR spectrum of MTPPBr. The peaks at 3.23 (d, 3H), and 7.63–7.79 (m, 15H) ppm are related to the CH3 hydrogens, and the three phenyl rings, respectively.
The 1H NMR of MTPPBr.
Figure 3 shows the 1H NMR spectrum of PCAT. The peaks at 6.77–7.34 (3H, aromatic), 9.31 (s, 1H), 9.68 (s, 1H), and 12.33 (s, 1H), ppm are related to the phenyl ring, the two OH groups and the OH of the acid group, respectively.
The 1H NMR of PCAT.
Figure 4 shows the 1H NMR spectrum of DES compound. The peaks at 3.15–3.19 (m, 6H) ppm are related to the two CH3 hydrogens, the peaks at 6.78–7.35 (m, 6H) ppm are related to the two phenyl ring of PCAT, the peaks at 7.75–7.90 (m, 30H) ppm are related to the six phenyl rings of MTPPBr, the peaks at 9.41–9.42 (s, 4H) are related to the four hydroxy of PCAT and the peak at 12.30 ppm (s, 2H) are related to the two hydroxy of acid groups. When DES is formed, the signal intensity of the active hydrogens weakens and shifts towards the low field. These observations indicate the hydrogen bond interactions between MTPPBr and PCAT,57 confirming the structure of the newly formed DES.
The 1H NMR of MTPPBr–PCAT–DES.
Figure 5 shows the 13C NMR spectrum of MTPPBr–PCAT–DES with seventeen peaks at 167.7, 150.4, 145.3, 135.2, 133.7, 133.5, 130.6, 130.4, 122.3, 122.1, 121, 191.6, 117, 116, 115.6, 8.27 and 7.37 ppm, which confirm the DES structure.
The 13C NMR of MTPPBr–PCAT–DES.
Figure 6 shows the 31P NMR spectrum of the DES catalyst which confirms the presence of the phosphorous in the structure of the molecule and its formation58.
The 31P NMR of MTPPBr–PCAT–DES.
To investigate the stability and thermal behavior of MTPPBr–PCAT–DES, the TGA–DTA analysis was performed (Fig. 7). The curve has two main breaks in the 389 °C and 432 °C regions. The first weight loss at about 389 °C is related to the removal of the acidic compound and the breakdown of the hydrogen bond. The last weight loss at about 432 °C is related to the breakdown of the ionic bonds.
The TGA–DTA curve of MTPPBr–PCAT.
However, according to the DTG, there are two small breaks in the area below 200 °C, which can be related to the very small amount of water released during the preparation of the catalyst, and can also be related to the noise of the device. If it is related to the release of water, according to the graph, this release of water is very little and no change has been made in the structure, and these results are inferred from the conducted 1HNMR analysis and the structure of the catalyst has not been damaged. These results indicate the high thermal stability of MTPPBr–PCAT.
Density is the thermophysical property most studied for DESs, and most of them are considered to have a density in the range of 1.0 and 1.35 g/cm3 at 298.15 K, which is higher than the density of water. So, a sample with a certain weight is mixed with a certain volume of water, then by using the formula (ρ = Pw/1 − mw/md), the density is calculated. The density of MTPPBr–PCAT–DES is about 1.2409 g/mL (Table 1)59.
To check the best ratio of MTPPBr to PCAT, the eutectic point experiment was performed, and different ratios of MTPPBr to PCAT were prepared. The eutectic point phase diagram (Fig. 8) showed that the best ratio for the novel DES formation is one mole of MTPPBr to one mole of PCAT. The melting points of MTPPBr and PCAT are 230 and 221 °C, respectively, but when a novel DES was prepared, the melting point decreased to 90 °C.
The eutectic points phase diagram of MTPPBr–PCAT–DES.
To optimize the preparation conditions of the target products, the reaction between barbituric acid, 4‐hydroxycoumarin, and benzaldehyde (synthesis of 2 h) was selected as a model reaction (Scheme 3). This reaction was evaluated using different solvents and the solvent-free condition, which the solvent-free condition was chosen as the suitable condition.
Synthesis of 2h by the novel DES catalyst.
Also, performing the model reaction was checked at different temperatures, and 80 °C was chosen as an appropriate temperature. Then, different amounts of the catalyst were used to find the optimum amount of the catalyst.
The best result was found to be the 1:1:1 mol ratio of barbituric acid, 4‐hydroxycoumarin, and benzaldehyde with 1.5 mmol of the novel DES catalyst at 80 °C in solvent-free conditions (Table 2).
Based on the obtained results from the model reaction (synthesis of 2h), 2(a-l) were synthesized from the reaction of barbituric acid, 4-hydroxycoumarin and aldehyde with 1.5 mmol of the DES catalyst at 80 °C in solvent-free conditions (Table 3). Short reaction times and high yields are the important features of this proposed method.
Light Yellow solid, M.P.: 255–258 °C; IR (KBr) ν = 3406, 3208, 3074, 2854, 1760, 1723, 1690, 1578, 1469, 1439, 1380, 1326, 1226, 1110, 791, 543 and 437 cm-1. 1H NMR (250 MHz, DMSO-d6) δ = 11.49 (s, 1H), 11.28 (s, 1H), 8.32–7.09 (m, 7H), 4.55 (s, 1H). 13C NMR (62.5 MHz, DMSO-d6) δ = 162.9, 161.3, 150.6, 148.8, 135.9, 134.5, 133.5, 131.8, 128.8, 127.0, 124.0, 122.7, 121.1, 116.2, 113.4, 105.3, 103.3, 100.6, 82.9, 31.7.; MS: m/z = 429.2 [M]+, base peak: m/z = 120.1.
Yellow solid, M.P.: 306–310 °C; IR (KBr) ν = 3224, 3142, 3073, 2991, 2839, 1740, 1696, 1541, 1655,1541,1496, 1390, 969, 767 and 524 cm-1. 1H NMR (250 MHz, DMSO-d6) δ = 11.28 (s, 1H), 11.16 (s, 1H), 7.87 (d, J = 8.1 Hz, 1H), 7.56 (t, J = 7.7 Hz, 1H), 7.36–7.29 (m, 2H), 7.08 (d, J = 8.5 Hz, 1H), 6.80–6.63 (m, 2H), 6.25 (s, 1H), 3.88–3.84 (m, 3H), 3.79 (s, 3H).13C NMR (62.5 MHz, DMSO-d6) δ = 165.8, 165.1, 164.4, 162.8, 155.9, 152.6, 150.6, 148.2, 132.1, 125.7, 124.3, 124.0, 119.3, 118.6, 117.2, 116.3, 111.5, 104.7, 83.8, 56.2, 55.9, 36.0.; MS: m/z = 420.3 [M]+, base peak: m/z = 276.1.
Red solid, M.P.: 250–253 °C; IR (KBr) ν = 3549, 3423, 3171, 3060, 2831, 1660, 1606, 1514, 1405, 1377, 1310, 1210, 944, 790, 529, 511 and 408 cm−1. 1H NMR (250 MHz, DMSO-d6) δ = 11.02 (s, 1H), 10.89 (s, 1H), 7.79 (d, J = 7.5 Hz, 1H), 7.49 (t, J = 7.6 Hz, 2H), 7.35 (d, J = 8.2 Hz, 1H), 7.24–7.18 (m, 4H), 6.26 (s, 1H), 3.44 (s, 6H). 13C NMR (62.5 MHz, DMSO-d6) δ = 168.0, 165.1, 163.1, 155.9, 154.6, 152.9, 150.7, 139.4, 131.5, 128.6, 124.5, 123.4, 120.4, 119.6, 116.0, 111.6, 109.9, 103.5, 91.6, 45.7, 40.0, 36.3.; MS: m/z = 403.3 [M]+, base peak: m/z = 259.1.
Crimson solid, M.P.: 242–246 °C; IR (KBr) ν = 3427, 3195, 3068, 2977, 1726, 1673, 1614, 1502, 1450, 1392, 1189, 1154, 792 and 514 cm-1. 1H NMR (250 MHz, DMSO-d6) δ = 10.98 (d, J = 11.6 Hz, 1H), 10.85 (d, J = 11.6 Hz, 1H), 9.03–5.78 (m, 9H), 3.48 (q, J = 6.9 Hz, 4H), 1.11 (q, J = 6.6 Hz, 6H). 13C NMR (62.5 MHz, DMSO-d6) δ = 168.1, 165.2, 163.1, 155.7, 152.6, 150.7, 139.9, 133.1, 131.5, 128.8, 124.3, 123.6, 121.9, 120.1, 116.7, 115.9, 111.3, 109.4, 105.1, 91.4, 44.6, 34.0, 12.9, 10.7.; MS: m/z = 431.6 [M]+, base peak: m/z = 272.1.
Yellow solid, M.P.: 219–222 °C; IR (KBr) ν = 3447, 3200, 3149, 3045, 2852, 1743, 1703, 1655, 1563, 1432, 1404, 1362, 1317, 1029, 930, 572, 517 and 410 cm-1. 1H NMR (250 MHz, DMSO-d6) δ = 11.32 (s, 1H), 11.23 (s, 1H), 7.87 (d, J = 7.7 Hz, 1H), 7.56 (d, J = 7.8 Hz, 1H), 7.30 (d, J = 8.6 Hz, 2H), 6.88 (s, 2H), 6.25 (d, J = 10.5 Hz, 1H), 6.00 (s, 1H).13C NMR (62.5 MHz, DMSO-d6) δ = 166.2, 164.6, 163.7, 162.5, 152.6, 151.6, 150.6, 141.6, 137.3, 132.2, 126.9, 124.4, 115.6, 113.2, 110.6, 106.2, 91.4, 31.9.; MS: m/z = 350.3 [M]+, base peak: m/z = 206.1.
White solid, M.P.: 295–298 °C; IR (KBr) ν = 3271, 3246, 3134, 2935, 2874, 1766, 1735, 1704, 1599, 1467, 1417, 1366, 1219, 1289, 1042, 965, 744, 538, 502 and 452 cm−1. 1H NMR (250 MHz, DMSO-d6) δ = 11.57 (s, 1H), 11.33 (s, 1H), 10.95 (s, 1H), 7.83–7.52 (m, 8H), 6.23 (s, 1H). 13C NMR (62.5 MHz, DMSO-d6) δ = 191.3, 169.6, 166.2, 162.8, 162.7, 151.6, 150.7, 148.2, 134.6, 134.6, 133.3, 129.4, 127.4, 125.7, 125.6, 123.2, 123.2, 83.4, 78.9, 51.1; MS: m/z = 403.2 [M]+, base peak: m/z = 231.1.
Light Yellow solid, M.P.: 350 > °C; IR (KBr) ν = 3422, 3206, 3093, 2943, 2841, 1743, 1606, 1573, 1439, 1406, 1306, 1209, 1025, 762 and 540 cm-1. 1H NMR (250 MHz, DMSO-d6) δ = 11.42 (s, 1H), 11.27 (s, 1H), 9.90 (s, 1H), 7.83 (d, J = 8.3 Hz, 2H), 7.73 (d, J = 7.8 Hz, 1H), 7.55 (d, J = 7.5 Hz, 1H), 7.32 (t, J = 7.9 Hz, 4H), 6.35 (s, 1H).
White solid, M.P.: 275–278 °C; IR (KBr) ν = 3400, 3213, 3059, 1727, 1655, 1621, 1494, 1450, 1296, 1195, 1031, 953, 798, 524 and 460 cm-1. 1H NMR (250 MHz, DMSO-d6) δ = 11.29 (d, J = 39.3 Hz, 2H), 8.28–7.09 (m, 9H), 6.02 (s, 1H).
White solid, M.P.: 256–259 °C; IR (KBr) ν = 3429, 3075, 2989, 2612, 1670, 1603, 1562, 1490, 1352, 1311, 1094, 907, 765 and 498 cm−1.
White solid, M.P.: 248–250 °C; IR (KBr) ν = 3427, 3208, 3086, 2844, 1752, 1701, 1672, 1443, 1573, 1296, 1219, 1192, 1095, 795, 763, 636, 567 and 423 cm−1.
Yellow solid, M.P.: 290–293 °C; IR (KBr) ν = 3406, 2945, 2732, 1655, 1618, 1529, 1494, 1347, 1312, 1186, 1101, 913, 793, 761, 720, 676 and 463 cm−1.
Yellow solid, M.P.: 290–293 °C; IR (KBr) ν = 3433, 3197, 3057, 2964, 1686, 1612, 1568, 1402 and 1188 cm−1.
The possible mechanism for the synthesis of 2(a-l) is shown in Scheme 4. First, barbituric acid is converted into its enol form by the novel DES catalyst and reacts with the DES-activated aldehyde with the deletion of an H2O molecule to form the intermediate (I). Then, intermediate (I) reacts as a Michael acceptor with 4‐hydroxycoumarin to form the intermediate (II). An intramolecular cyclization and deletion of a water molecule will change the intermediate (II) into the final product59.
Proposed mechanism for the synthesis of 2(a-l).
To prove the efficiency of the novel DES catalyst, its recyclability was tested in four consecutive runs. Therefore, after completion of the model reaction, ethanol and water were added, and the reaction mixture filtered to separate the catalyst. The DES catalyst was separated from the filtrate by removing the ethanol and water solvents and used in next three successive runs with the similar reaction conditions. The results show relatively no significant loss of activity (95%, 93%, 89%, and 83%, respectively) (Table 4, Fig. 9).
Reusability of the MTPPBr–PCAT–DES catalyst in four consecutive reaction runs.
Also, the 1H NMR, 13C NMP, and 31P NMR spectra of the used DES are very similar to the corresponding fresh one, indicating preservation of the DES structure during the reactions (Figs. 10, 11, 12).
The 1H NMR of recovered catalyst.
The 13C NMR of recovered catalyst.
The 31P NMR of recovered catalyst.
Table 5 shows the comparison of different methods for the synthesis of 2(a-l) with our proposed procedure.
For example, 2h was synthesized by trisodium citrate in EtOH:H2O (1:1 v/v) with 83% yield in 45 min60. Also, it was synthesized by an electrocatalytic method and tetra-n-butyl-ammonium fluoride (TBAF) in PrOH with 54% yield in 45 min55. This comparison reveals the advantage of our proposed method with performing the reaction in shorter reaction time and higher yields.
In summary, a novel deep eutectic solvent (MTPPBr–PCAT–DES) was prepared from one mole of MTPPBr and one mole of PCAT (according to the eutectic point phase diagram) to expand the field of green and environmentally friendly catalysts, and the structure of the novel DES compound was confirmed by various techniques. Synthesis of deep eutectic mixtures is more energy efficient and can be synthesized simply by mixing and heating the components without purification. DESs can be effective not only as moderate, cheap, and environmentally safe solvents but also as recoverable and reusable organic catalysts to facilitate organic transformations.
Then, the novel DES was used as an efficient, powerful, and recyclable catalyst in the synthesis of pyranopyrimidines with short reaction times and high yields.
All data generated or analyzed during this study are included in this published article and its Supplementary Information files.
Dindarloo Inaloo, I., Majnooni, S. & Esmaeilpour, M. Superparamagnetic Fe3O4 nanoparticles in a deep eutectic solvent: An efficient and recyclable catalytic system for the synthesis of primary carbamates and monosubstituted ureas. Eur. J. Org. Chem. 2018, 3481–3488 (2018).
Anastas, P. & Eghbali, N. Green chemistry: Principles and practice. Chem. Soc. Rev. 39, 301–312 (2010).
CAS PubMed Google Scholar
Poliakoff, M., Fitzpatrick, J. M., Farren, T. R. & Anastas, P. T. Green chemistry: Science and politics of change. Science 297, 807–810 (2002).
ADS CAS PubMed Google Scholar
Constable, D. J., Curzons, A. D. & Cunningham, V. L. Metrics to ‘green chemistry which are the best?. Green Chem. 4, 521–527 (2002).
CAS Google Scholar
Newman, S. G. & Jensen, K. F. The role of flow in green chemistry and engineering. Green Chem. 15, 1456–1472 (2013).
CAS Google Scholar
Abbott, A. P., Capper, G., Davies, D. L., Munro, H. L., Rasheed, R. K. & Tambyrajah, V. Preparation of novel, moisture-stable, Lewis-acidic ionic liquids containing quaternary ammonium salts with functional side chains electronic supplementary information (ESI) available: plot of conductivity vs. temperature for the ionic liquid formed from zinc chloride and choline chloride (2∶ 1). Chem. Commun. 2010–2011 (2001).
Marset, X., Pérez, J. M. & Ramón, D. J. Cross-dehydrogenative coupling reaction using copper oxide impregnated on magnetite in deep eutectic solvents. Green Chem. 18, 826–833 (2016).
CAS Google Scholar
Zhang, Q., Vigier, K. D. O., Royer, S. & Jérôme, F. Deep eutectic solvents: Syntheses, properties and applications. Chem Soc Rev. 41, 7108–7146 (2012).
CAS PubMed Google Scholar
Dai, Y., Van Spronsen, J., Witkamp, G. J., Verpoorte, R. & Choi, Y. H. Ionic liquids and deep eutectic solvents in natural products research: Mixtures of solids as extraction solvents. J. Nat. Prod. 76, 2162–2173 (2013).
CAS PubMed Google Scholar
Dindarloo Inaloo, I. & Majnooni, S. Carbon dioxide utilization in the efficient synthesis of carbamates by deep eutectic solvents (DES) as green and attractive solvent/catalyst systems. New J. Chem. 43, 11275–11281 (2019).
CAS Google Scholar
Alavi, L., Seidi, S., Jabbari, A. & Baheri, T. Deep eutectic liquid organic salt as a new solvent for carrier-mediated hollow fiber liquid phase microextraction of lead from whole blood followed by electrothermal atomic absorption spectrometry. New J. Chem. 41, 7038–7044 (2017).
CAS Google Scholar
Dindarloo Inaloo, I. & Majnooni, S. Deep eutectic solvents (des) as green and efficient solvent/catalyst systems for the synthesis of carbamates and ureas from carbonates. ChemistrySelect 4, 7811–7817 (2019).
CAS Google Scholar
Bagherzadeh, N., Sardarian, A. R. & Dindarloo Inaloo, I. Green and efficient synthesis of thioureas, ureas, primary O-thiocarbamates, and carbamates in deep eutectic solvent/catalyst systems using thiourea and urea. New J Chem. 45, 11852–11858 (2021).
CAS Google Scholar
Lindberg, D., de la Fuente Revenga, M. & Widersten, M. Deep eutectic solvents (DESs) are viable cosolvents for enzyme-catalyzed epoxide hydrolysis. J. Biotechnol. 147, 169–171 (2010).
CAS PubMed Google Scholar
Wagle, D. V., Zhao, H. & Baker, G. A. Deep eutectic solvents: Sustainable media for nanoscale and functional materials. Acc. Chem. Res. 47, 2299–2308 (2014).
CAS PubMed Google Scholar
Nguyen, H. T., Chau, D. K. N. & Tran, P. H. A green and efficient method for the synthesis of pyrroles using a deep eutectic solvent ([CholineCl][ZnCl2]3) under solvent-free sonication. New J Chem. 41, 12481–12489 (2017).
Alonso, D. A. et al. Deep eutectic solvents: The organic reaction medium of the century. EurJOC. 2016, 612–632 (2016).
CAS Google Scholar
Batalha, PN Recent Advances in Multicomponent Reactions: A Perspective between the years 2008 and 2011. Rev. Virtual Quim.4, 13–45 (2012).
CAS Google Scholar
Horvath, I. T. & Anastas, P. T. Innovations and green chemistry. Chem. Rev. 107, 2169–2173 (2007).
CAS PubMed Google Scholar
Biggs-Houck, J. E., Younai, A. & Shaw, J. T. Recent advances in multicomponent reactions for diversity-oriented synthesis. Curr. Opin. Chem. 14, 371–382 (2010).
CAS Google Scholar
Rezayati, S. et al. Magnetic silica-coated picolylamine copper complex [Fe3O4@SiO2@gp/picolylamine–Cu(ii)]-catalyzed biginelli annulation reaction. Inorg. Chem. 61, 992–1010 (2021).
PubMed Google Scholar
Rezayati, S., Ramazani, A., Sajjadifar, S., Aghahosseini, H. & Rezaei, A. Design of a Schiff base complex of copper coated on epoxy-modified core–shell MNPs as an environmentally friendly and novel catalyst for the one-pot synthesis of various chromene-annulated heterocycles. ACS Omega. 6, 25608–25622 (2021).
CAS PubMed PubMed Central Google Scholar
Hosseinzadeh, Z., Ramazani, A. & Razzaghi-Asl, N. Anticancer nitrogen-containing heterocyclic compounds. Curr. Org. Chem. 22, 2256–2279 (2018).
CAS Google Scholar
Ramazani, A. & Kazemizadeh, A. R. Preparation of stabilized phosphorus ylides via multicomponent reactions and their synthetic applications. Curr. Org. Chem. 15, 3986–4020 (2011).
CAS Google Scholar
Kalantari, F., Rezayati, S., Ramazani, A. & Poor Heravi, M. Syntheses and structures of magnetic nanodendrimers and their catalytic application in organic synthesis. Appl. Organomet. Chem. 37, e7064 (2023).
CAS Google Scholar
Khoobi, M. et al. Coumarin-based bioactive compounds: Facile synthesis and biological evaluation of coumarin-fused 1,4-thiazepines. Chem. Biol. Drug Des. 78, 580–586 (2011).
CAS PubMed Google Scholar
Rouhani, M., Ramazani, A. & Joo, S. W. Novel, fast and efficient one-pot sono-chemical synthesis of 2-aryl-1,3,4-oxadiazoles. Ultrason. Sonochem. 21, 262–267 (2014).
CAS PubMed Google Scholar
Ali, A. Q. et al. Synthesis, structure and in vitro anticancer, DNA binding and cleavage activity of palladium(II) complexes based on isatin thiosemicarbazone derivatives. Appl. Organomet. Chem. 31, e3813 (2017).
Souldozi, A., Ramazani, A., Dadrass, A. R., Ślepokura, K. & Lis, T. Efficient one-pot synthesis of alkyl 2-(dialkylamino)-4-phenylthiazole-5-carboxylates and single-crystal X-ray structure of methyl 2-(diisopropylamino)-4-phenylthiazole-5-carboxylate. Helv. Chim. Acta. 95, 339–348 (2012).
CAS Google Scholar
Ramazani, A., Mahyari, A. T., Rouhani, M. & Rezaei, A. A novel three-component reaction of a secondary amine and a 2-hydroxybenzaldehyde derivative with an isocyanide in the presence of silica gel: An efficient one-pot synthesis of benzo[b]furan derivatives. Tetrahedron Lett. 50, 5625–5627 (2009).
CAS Google Scholar
Aghahosseini, H. et al. Vinylphosphonium salt-mediated reactions: A one-pot condensation approach for the highly cis-selective synthesis of N-benzoylaziridines and the green synthesis of 1,4,2-dioxazoles as two important classes of heterocyclic compounds. Org. Lett. 21, 22–26 (2019).
CAS PubMed Google Scholar
Bagley, M. C. et al. Microwave-assisted synthesis of pyrimidine libraries. QSAR Comb. Sci. 23, 859–867 (2004).
CAS Google Scholar
Kamdar, N. R., Haveliwala, D. D., Mistry, P. T. & Patel, S. K. Design, synthesis and in vitro evaluation of antitubercular and antimicrobial activity of some novel pyrano-pyrimidines. Eur. J. Med. Chem. 45, 5056–5063 (2010).
CAS PubMed Google Scholar
Grivaky, E. M. & Lee, S. Synthesis and antitumor activity of 2,4-diamino-6-(2,5-di-methoxybenzyl)-5-methylpyrido[2,3-d]pyrimidine. J. Med. Chem. 23, 327–329 (1980).
Valderrama, J. A. et al. Studies on quinones Part 44: Novel angucyclinone N-heterocyclic analogues endowed with antitumoral activity. Bioorg. Med. Chem. 16, 10172–10181 (2008).
CAS PubMed Google Scholar
Harbone J. B. (ed.) The Flavonoids Advances in Research, since 1980 (Chapman & Hall, London).
Iacobucci, G. A. & Sweeny, J. G. The chemistry of anthocyanins, anthocyanidins and related flavylium salts. Tetrahedron 39, 3005–3038 (1983).
CAS Google Scholar
Bohm, B. A., Choy, J. B. & Lee, A. Y. M. Flavonoids of Balsamorhiza and Wyethia. Phytochemistry 28, 501–503 (1989).
CAS Google Scholar
Moro, A. J. et al. 2,2′-spirobis[chromene] derivatives, chemistry, and their relation with the multistate system of anthocyanins. J. Org. Chem. 82, 5301–5309 (2017).
CAS PubMed Google Scholar
Parmar, V. S. et al. Phytochemistry of the genus Piper. Phytochem. 46, 597–673 (1997).
CAS Google Scholar
Polyakov, V. V. Chemical modification of the natural flavonoid myricetin. Chem. Nat. Compd. 35, 21–28 (1999).
CAS Google Scholar
Grivsky, E. M., Lee, S., Sigel, C. W., Duch, D. S. & Nichol, C. A. Synthesis, and antitumor activity of 2,4-diamino-6-(2,5-dimethoxybenzyl)-5-methylpyrido[2,3-d]-pyrimidine. J. Med. Chem. 23, 327–329 (1980).
CAS PubMed Google Scholar
Esmaeilia, A. A., Salehan, F., Habibi, A. & Fakhari, A. R. Efficient synthesis of novel pyrano[2,3-d]pyrido[1,2-a]pyrimidines via isocyanide-based three-component reaction. Tetrahedron Lett. 57, 100–102 (2016).
Alizadeh, A., Ghanbaripour, R. & Ng, S. W. An efficient synthesis of chromeno[2,3-d]- pyrimidine-2,4-diones with a nitroketene-aminal moiety at C(5) by a one-pot four-component reaction. Helv. Chim. Acta. 98, 663–667 (2015).
CAS Google Scholar
Narayana Maddila, S., Maddila, S., van Zyl, W. E. & Jonnalagadda, S. B. Mn doped ZrO2 as a green, efficient and reusable heterogeneous catalyst for the multicomponent synthesis of pyrano[2,3-d]pyrimidines. RSC Adv. 5, 37360–37366 (2015).
ADS Google Scholar
Brahmachari, G. & Banerjee, B. Facile and one-pot access to diverse and densely functionalized 2-amino-3-cyano-4H-pyrans and pyran-annulated heterocyclic scaffolds via an eco-friendly multicomponent reaction at room temperature using urea as a novel organo-catalyst. ACS Sustain. Chem. Eng. 2, 411–422 (2014).
CAS Google Scholar
Ghorbani-Vaghei , R. , Maghbooli , Y. , Mahmoodi , J. & Shahriari , A. Poly(N-bromo-N-ethylbenzene-1,3-disulfonamide) and N, N, N′, N′-tetrabromobenzene- . 1,3-disulfonamides as new efficient reagents for one-pot synthesis of furans and pyranopyrimidi-nones (thiones).RSC Adv.5, 74336–74341 (2015).
ADS CAS Google Scholar
Fan, X. et al. Tandem reactions leading to bicyclic pyrimidine nucleosides and benzopyran-4-ones. J. Org. Chem. 76, 982–985 (2011).
CAS PubMed Google Scholar
Rajesh, N. & Prajapati, D. A copper-catalyzed one-pot three-component tandem conjugative alkynylation/6-endo cyclization sequence: Access to pyrano[2,3-d]pyrimi-dines. Org. Biomol. Chem. 13, 4668–4672 (2015).
CAS PubMed Google Scholar
Valderrama, J. A. et al. Studies on quinones. Part 44: Novel angucyclinone N-heterocyclic analogues endowed with antitumoral activity. Bioorg. Med. Chem. 16, 10172–10181 (2008).
CAS PubMed Google Scholar
Heber, D., Heers, C. & Ravens, U. Positive inotropic activity of 5-amino-6-cyano-1,3-dimethyl-1,2,3,4-tetrahydropyrido[2,3-d]pyrimidine-2,4-dione in cardiac muscle from guinea-pig and man. Part 6: Compounds with positive inotropic activity. Die Pharm. 48, 537–541 (1993).
CAS Google Scholar
Furuya, S., Ohtaki. T. Eur. Pat. Appl. EP. 608565, 1994, Chem. Abstr. 121, 205395 (1994).
Debbabi, M. et al. Design and synthesis of novel potent anticoagulant and anti-tyrosinase pyrano-pyrimidines and pyranotriazolopyrimidines: Insights from molecular docking and SAR analysis. Bioorg. Chem. 82, 129–138 (2019).
CAS PubMed Google Scholar
Lee , JH , Bang , HB , Han , SY & Jun , JG An efficient synthesis of (+)-decurcinol from umbelliferone.Tetrahedron Lett.Rev. 48, 2889–2892 (2007).
CAS Google Scholar
Kazemi-Rad, R. A green approach to electrosynthesis of chromeno[3’,4’:5,6]pyrano- [2,3-d]pyrimidines. Anal. Methods Environ. Chem. J. 1, 39–46 (2018).
Kazemi-Rad, R., Azizian, J. & Kefayati, H. Electrogenerated acetonitrile anions/tetra-butylammonium cations: An effective catalytic system for the synthesis of novel chrom- eno[3′,4′:5,6]pyrano[2,3-d]pyrimidines. Tetrahedron Lett. 55, 6887–6890 (2014).
CAS Google Scholar
Chen, W. et al. Tailoring hydro-phobic deep eutectic solvent for selective lithium recovery from the mother liquor of Li2CO3. J. Chem. Eng. 420, 127648 (2021).
CAS Google Scholar
Cooper, M. K., Downes, J. M., Duckworth, P. A. & Tiekink, E. R. T. Preparation of hybrid bidentate phosphine-ligands by reduction of their benzylphosphonium or phenylphosphonium salts-X-ray crystal-structure of 2-aminophenyltriphenyl-phosphonium tetrachloronickelate(II). Aust. J. Chem. 45, 595–609 (1992).
CAS Google Scholar
Wang, H., Jing, Y., Wang, X., Yao, Y. & Jia, Y. Ionic liquid analogous formed from magnesium chloride hexahydrate and its physicochemical properties. J. Mol. Liq. 163, 77–82 (2011).
CAS Google Scholar
Brahmachari, G. & Nurjamal, K. Ultrasound-assisted and trisodium citrate dihydrate-catalyzed green protocol for efficient and one-pot synthesis of substituted chromeno- [3′,4′:5,6]pyrano[2,3-d]pyrimidines at ambient conditions. Tetrahedron Lett. 60, 1904–1908 (2019).
CAS Google Scholar
The authors are grateful to the Bu-Ali Sina University for the support of this work.
Department of Organic Chemistry, Faculty of Chemistry, Bu-Ali Sina University, Hamedan, 6517838683, Iran
Arezo Monem, Davood Habibi & Hadis Goudarzi
You can also search for this author in PubMed Google Scholar
You can also search for this author in PubMed Google Scholar
You can also search for this author in PubMed Google Scholar
A.M. did the lab experiment. D.H. wrote the main manuscript text. H.G. is a co-author. All authors reviewed the manuscript.
The authors declare no competing interests.
Springer Nature remains neutral with regard to jurisdictional claims in published maps and institutional affiliations.
Open Access This article is licensed under a Creative Commons Attribution 4.0 International License, which permits use, sharing, adaptation, distribution and reproduction in any medium or format, as long as you give appropriate credit to the original author(s) and the source, provide a link to the Creative Commons licence, and indicate if changes were made. The images or other third party material in this article are included in the article's Creative Commons licence, unless indicated otherwise in a credit line to the material. If material is not included in the article's Creative Commons licence and your intended use is not permitted by statutory regulation or exceeds the permitted use, you will need to obtain permission directly from the copyright holder. To view a copy of this licence, visit http://creativecommons.org/licenses/by/4.0/.
Monem, A., Habibi, D. & Goudarzi, H. An acid-based DES as a novel catalyst for the synthesis of pyranopyrimidines. Sci Rep 13, 18009 (2023). https://doi.org/10.1038/s41598-023-45352-4
DOI: https://doi.org/10.1038/s41598-023-45352-4
Anyone you share the following link with will be able to read this content:
Sorry, a shareable link is not currently available for this article.
Provided by the Springer Nature SharedIt content-sharing initiative
By submitting a comment you agree to abide by our Terms and Community Guidelines. If you find something abusive or that does not comply with our terms or guidelines please flag it as inappropriate.
Scientific Reports (Sci Rep) ISSN 2045-2322 (online)
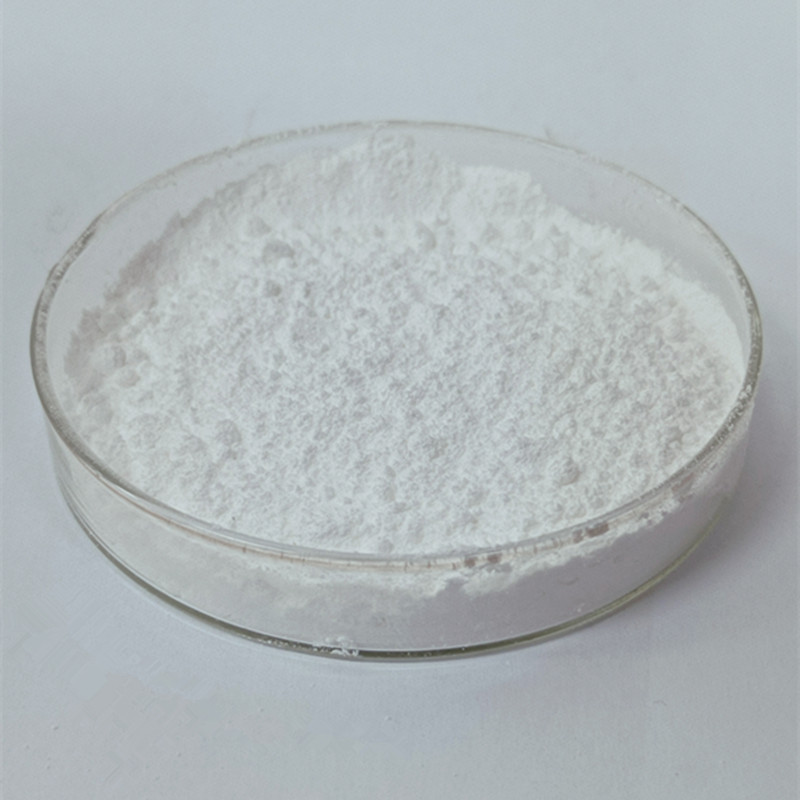
Solid Acid Catalyst Sign up for the Nature Briefing newsletter — what matters in science, free to your inbox daily.