Thank you for visiting nature.com. You are using a browser version with limited support for CSS. To obtain the best experience, we recommend you use a more up to date browser (or turn off compatibility mode in Internet Explorer). In the meantime, to ensure continued support, we are displaying the site without styles and JavaScript.
Scientific Reports volume 13, Article number: 10994 (2023 ) Cite this article Cjx2 Contactor

An Author Correction to this article was published on 24 July 2023
This article has been updated
The release of excessive carbon dioxide (CO2) into the atmosphere poses potential threats to the well-being of various species on Earth as it contributes to global working. Therefore, it is necessary to implement appropriate actions to moderate CO2 emissions. A hollow fiber membrane contactor is an emerging technology that combines the advantages of separation processes and chemical absorptions. This study investigates the efficacy of wet and falling film membrane contactors (FFMC) in enhancing CO2 absorption in a monoethanolamine (MEA) aqueous solution. By analyzing factors such as membrane surface area, gas flow rate, liquid inlet flow rates, gas–liquid contact time, and solvent loading, we examine the CO2 absorption process in both contactors. Our results reveal a clear advantage of FFMC, achieving an impressive 85% CO2 removal efficiency compared to 60% with wet membranes. We employ COMSOL Multiphysics 6.1 simulation software and finite element analysis to validate our findings, demonstrating a close agreement between predicted and experimental values, with an average relative error of approximately 4.3%. These findings highlight the significant promise of FFMC for applications in CO2 capture.
The mass transfer and hydrodynamic performance of separation devices used in gas absorption processes are influenced by several crucial factors. These factors include the gas–liquid contact area, mass transfer coefficients, and pressure drop1. Membrane contactors (MCs) gain attention in pilot and industrial scales, particularly for carbon capture2, reducing energy consumption and cost. Challenges include pore wetting, solvent selection, and fouling with potential solutions. The review highlights working principles, comparisons with gas separation, module designs, and commercial implementations3,4,5. They consist of a porous membrane that acts as a physical barrier between the gas and liquid phases, allowing selective transfer of gases while preventing the mixing of the two phases. This unique structure enables several advantages regarding mass transfer rates and process efficiency6. MCs offer a substantial interfacial area, promoting the efficient mass transfer and enabling the effective removal of specific impurities like CO2 and H2S from gas streams.
Furthermore, MCs demonstrate low-pressure drops, decreasing energy consumption and operating costs7,8. MCs have been explored to replace traditional packed columns in carbon capture and sulfur dioxide removal applications9. Overall, the use of MCs represents a promising approach for gas–liquid mass transfer in various industries, and further research is needed to optimize their performance and commercial viability10,11,12,13. Hydrophobic polymer materials such as polypropylene, polytetrafluoroethylene, and polyvinylidene fluoride are the most common hydrophobic membranes used in CO2 absorption processes14. Membrane contactors, which are made up of several hollow fibers assembled, have a large specific surface area, providing a high interface area for gas–liquid contact15,16,17,18. In the membrane absorption process using hydrophobic membranes, the gas to be absorbed first transfers from the bulk gas phase to the gas-membrane boundary and then diffuses through the membrane pores to the gas–liquid interface where the absorption occurs19. The overall mass transfer process involves three resistances in series: the gas phase resistance, the membrane resistance, and the liquid resistance. Ideally, the membrane pores are filled with gas and reject liquid permeation to ensure low mass transfer resistance. However, most polymeric membranes used in membrane absorption are prone to wetting over prolonged periods of operation, which can negatively impact their performance20.
Membrane wetting can occur for various reasons, including non-optimal material properties such as inadequate hydrophobicity, wrong pore size, low chemical resistance, and operational issues such as liquid evaporation and condensation21. As a result, wetting of membrane pores is a significant technical challenge for membrane contactors, as it can significantly increase mass transfer resistance22,23. While some efforts have been made to improve material hydrophobicity and increase permeability by using asymmetric membrane structures to reduce pore wetting, it is still an ongoing issue. Alternatively, absorption processes that do not rely on hydrophobic pore-based membranes may be considered24. Using a hydrophilic porous membrane for CO2 absorption into aqueous solutions in a falling liquid-film mode is a novel approach that shows promise25. In this mode, the feed liquid permeates through the hydrophilic porous membrane pores, forming a falling liquid film on the surface of the permeation side of the membrane due to gravity. The thin liquid film can contact the gas fluid in a co- or counter-current flow26. In theory, the falling liquid film should completely cover the surface of the permeate side of a hydrophilic porous membrane27. As a result, the falling liquid-film approach offers the benefits of a high gas–liquid contact area while eliminating mass transfer resistance in the pores, making it an attractive alternative to hydrophobic pore-based membrane absorption28,29. Ceramic membranes have excellent mechanical strength, chemical stability, and thermal stability, making them resistant to corrosive amine solutions used for CO2 absorption21,30,31,32. Overall, ceramic membranes are well-suited for the falling liquid-film CO2 absorption process involving aqueous amine solutions33,34,35,36.
Several mathematical models were developed to depict the transport of gas impurities such as CO2 and H2S within a solvent across a hollow fiber membrane contactor14,15,16,17,18,31,37,38. A comprehensive model was developed to describe the gas and liquid reactions and transport inside the contactor under wetting or non-wetting conditions39. To the author's knowledge, no models have been developed thus far to comprehensively describe mass transport in a falling film membrane contactor. This study specifically examined the modeling and simulation of CO2 absorption using wet and falling liquid-film membrane contactors. Porous ceramic hollow hydrophilic membranes were employed in contactors with an aqueous monoethanolamine (MEA) solution. The research thoroughly compared wet membranes and falling film membrane contactors while exploring different operating parameters.
The dynamic model that has been created depicts a two-dimensional simulation of the process of CO2 absorption from a gaseous mixture (CO2/N2) into an aqueous solvent of MEA, which occurs within a wet membrane mode, and a falling liquid film, membrane contactor (as shown in Fig. 1). The process involves solvent diffusion across the membrane walls, creating a thin solvent film. The solvent film is designed to selectively absorb CO2 from a gas mixture of 10% CO2; the remainder is N2.
Schematic diagram describes solvent diffusion across membrane walls, forming a wet membrane (wet mode) and a thin falling film (falling film) to absorb CO2 from gas mixtures containing 10% CO2/N2. Figure generated using Microsoft Visio Professional 2016 (Microsoft.com).
The ceramic membrane used in this process is hydrophilic and includes an inner space called the lumen that the solvent travels through before reaching the membrane walls40. Once the solvent reaches the outside of the walls, it forms a thin film. The solvent film absorbs the CO2 from the inert ga moving in the opposite direction. As the inert gas flows, it creates a concentration gradient that causes the CO2 to be absorbed into the solvent as it passes near the solvent film. This method is commonly employed in various applications, including carbon capture, where the isolation of CO2 from other gases is necessary. By utilizing a ceramic membrane and solvent diffusion, it is possible to selectively extract CO2 from a gas stream, resulting in a cleaner gas product.
The falling film results from the diffusion of the amine solvent through the wet membrane porous to the gas side. In developing the transient model, the following assumptions are considered38:
Isothermal, steady state operating conditions.
The membrane is thoroughly wet.
Henry's law governs the gas–liquid equilibrium constant.
The gas is in ideal condition.
The velocity profile of the liquid in the tube and the inert gas in the shell are laminar.
Based on the steady-state assumption, the component material balance inside the tube (\({C}_{i,t}\) ), taking into consideration the convective term, starts with velocity on the tube side (\({V}_{z,t}\) ), diffusion term began with diffusion coefficient (\({D}_{i,t}\) ), and reaction terms (\({R}_{i}\) ) can be represented by Eq. (1):
The average velocity (\({V}_{av}\) ) and the ratio of the variable radius (\(r\) ) to the inner radius of the inner tube (\({r}_{1}\) ) are used to represent the velocity profile on the tube side, as depicted by the following parabola. The Navier–Stocks equation is also applied, revealing notable variations in the velocity profile:
Inside the membrane segment, due to the assumption of wetted mode, the membrane pores are full of solvent, and diffusion starts with \({D}_{i,m}\) , and reactions are the only governing equations and the convective term is neglected, accordingly the concentration profile in the membrane pores (\({C}_{i,m}\) ) represents the material component balance as follows:
For the case solvent in the tube side and gas in the shell side, for a dry membrane (non-wetted), the diffusion coefficient of CO2 in the membrane pores (\({D}_{i,m}\) ) is determined by porosity (\(\epsilon\) ), tortuosity (\(\tau\) ) of the porous membrane, and the diffusion of the component in the shell side (\({D}_{i,s}\) ):
Based on the solvent flowing in the tube side, for a wet membrane (total wetting), the diffusion coefficient of CO2 in the membrane pores (\({D}_{i,wm}\) ) is determined by porosity (\(\epsilon\) ), tortuosity (\(\tau\) ) of the porous membrane, and the diffusion of the component in the tube side (\({D}_{i,t}\) ):
The material balance governing the equation of existing components in the falling film is represented by the diffusion coefficient in the liquid film (\({D}_{i,f}\) ), the concentration of the component on the film side (\({C}_{i,f}\) ), and the reaction rate \(({R}_{i,f})\) , the film velocity can be neglected because it is prolonged:
The concentration profile of carbon dioxide (\({C}_{i,s})\) in the shell side is represented by the diffusion coefficient of CO2 on the shell side (\({D}_{i,s}\) ), and the velocity profile on the shell side (\({V}_{z,s}\) ), no reaction rate occurs on the side except in the falling film:
The Navier–Stokes equations regulate the flow of liquids and can be considered Newton's second law of motion for fluids. For a compressible Newtonian fluid, this results in the following:
where "u" represents the velocity of the fluid, "p" represents the pressure of the fluid, "ρ" represents the density of the fluid, and "μ" represents the fluid's dynamic viscosity.
The separate terms refer to the inertial, pressure, viscous, and external forces acting on the fluid. The parameters used in the model development are shown in Table 1.
The physical and chemical properties used in the modeling are shown in Table 2. The porosity of a hollow fiber ceramic membrane can vary depending on the specific membrane and its intended use. However, in general, the porosity of a hollow fiber ceramic membrane can range from around 30 to 50%41.
When considering the reaction kinetics, it is possible to express it as a second-order reaction involving the first order of each reactant species. By utilizing the following formula, the reaction rate can be determined at various temperatures46:
We used a computer program called COMSOL Multiphysics version 6.1® to create a model of the transport of CO2 through a tube side, wet membrane, falling film, and shell side. The model used a Cartesian coordinate system to estimate the CO2 concentration at different points in the system. In order to discretize the computational domain, we are using structured multi-block grids, with the densest mesh being used in the region where the concentration gradient is largest and the flow phenomenon is most complex (presumably near the liquid film), and the loosest mesh being used in the shell side region where the velocity change is smallest and the flow phenomenon is simplest. After the meshing process is completed, we end up with a total of around 55,000 meshes. This information is shown in Fig. 2, which presumably provides a visual representation of the meshing scheme.
Optimum meshing of falling film membrane contactor domains generated using Comsol Multiphysics 6.1. Image generated using Comsol Multiphysics version 6.1 (comsol.com).
Figure 3 shows the mesh analysis approach used in the simulation and calculation of the CO2 removal fraction in the falling film membrane contactor. The CO2 removal efficiency does not change significantly when the number of mesh exceeds fifty thousand.
Simulation of CO2 removal fraction in the falling film membrane contactor conducted using COMSOL 6.1 software, considering the number of mesh elements used for discretization.
Figure 4 compares mathematical model predictions with experimental data for CO2 removal efficiency and removal flux as a function of inlet gas feed rate. The results indicate that as the inert gas flow rate increases, the CO2 removal fraction decreases while the CO2 adsorption rate increases47. A higher gas flow rate reduces the amount of time CO2 spends in the membrane contactor, leading to a reduction in CO2 removal efficiency. On the other hand, a higher gas flow rate provides more CO2 for absorption, increases turbulence, and decreases the gas-side mass transfer resistance, contributing to an increase in the CO2 absorption rate. However, this increase slows as the gas flow rate rises because the proportion of resistance from gas phase mass transfer decreases, and the process becomes dominated by liquid-film control23. The model's predictions agree with the experimental data, demonstrating its reliability and making it a valuable tool for probing other parameters.
Comparing model predictions of CO2 removal flux and efficiency against gas flow rate in a falling film membrane contactor with the corresponding experimental data27.
Figure 5 is a 2D surface plot that illustrates the changes in the concentration of CO2 across the membrane module, where the concentration of CO2 on the membrane shell side decreases due to absorption by the solvent film. This absorption mechanism is commonly used in gas separation processes, which creates a concentration gradient across the membrane, driving the passage of CO2 from the feed gas stream to the solvent. As a result, the concentration of CO2 in the feed stream decreases as it passes through the membrane module, while the concentration of CO2 in the permeate solvent stream increases48. The total flux arrows indicate the direction of CO2 transport through the shell side, eventually absorbed in the thin solvent film. In this case, the solvent film absorbs CO2, creating a concentration gradient across the falling liquid films. The concentration gradient created by the absorption of CO2 by the solvent film drives the passage of CO2 from the feed gas stream to the wetted membrane falling film49. However, CO2 concentration in the permeate stream is increasing, indicating that the solvent is capturing and transferring more CO2 through the wetted membrane film50.
The surface plot of the CO2 concentration profile across the membrane module at a gas feed rate is 100 ml/min and a liquid feed rate of 60 ml/min. The image was generated using Comsol Multiphysics version 6.1 (comsol.com).
Figure 6 demonstrates that increasing the liquid flow rate improves the ability to absorb CO2 and absorption efficiency. This is due to two primary reasons: a higher liquid flow rate results in more active MEA molecules, enhancing absorption capacity and reaction kinetics. Secondly, the increased turbulence of the falling liquid film boosts the mass transfer coefficient, which is essential for liquid phase-controlled mass transfer. Nevertheless, avoiding excessively increasing the liquid flow rate is crucial since the absorption flux reaches a plateau51. The point at which the absorption flux reaches a plateau depends on the specific absorption process and the properties of the absorbent solution. It is essential to optimize the liquid flow rate to achieve the highest possible absorption efficiency without exceeding the point where the absorption flux plateaus52.
Effect of solvent feed rate on the absorption removal efficiency and removal flux of carbon dioxide from an inert gas (10% CO2/N2) in a falling film membrane contactor.
The typical concentration of monoethanolamine (MEA) in carbon dioxide (CO2) absorption processes can vary depending on the application and operating conditions. However, the typical range is around 20–30 weight percent (wt%) MEA53. Higher MEA concentrations can increase the solubility of CO2 in the solvent and improve the CO2 removal efficiency, but they also increase the energy consumption and cost associated with the regeneration process.
Figure 7 shows that the effect of carbon dioxide (CO2) loading in aqueous monoethanolamine (MEA) on the CO2 fraction removal fraction and flux from simulated flue gas can be significant. MEA is commonly used as a solvent in chemical absorption processes for capturing CO2 from flue gas, biogas, and natural gas. As the CO2 loading in the MEA solution increases, the CO2 removal efficiency and flux typically decrease54. This is because when the concentration of CO2 increases, it becomes less soluble in the MEA solution, which reduces the driving force for CO2 absorption. Moreover, when there is a higher CO2 loading, solid particles, also known as "MEA salts", can form, lowering the CO2 removal efficiency and increasing the pressure drop in the absorber55. To maintain a high CO2 removal efficiency and flow rate, it is necessary to regularly regenerate the MEA solution to remove the absorbed CO2 and maintain a low CO2 concentration. Regenerating the MEA solution involves heating it to release the CO2, which is then typically compressed and stored for later use or permanent disposal. Controlling the CO2 loading in the MEA solution is essential for effective CO2 capture while minimizing the energy consumption and cost of the regeneration process when capturing CO2 from natural gas or flue gas56.
Effect of CO2 loading in the MEA aqueous solution on the CO2 fraction removal and removal flux for a falling film membrane contactor. Gas feed rate 100 ml/min.
Figure 8 demonstrates how the gas feed rate affects the CO2 removal fraction and flux for the falling film and wet-membrane cases. In both cases, as the gas flow rate increases, the CO2 removal efficiency decreases because the gas has less time to dissolve the CO2 in the liquid film due to the reduced contact duration. Moreover, a higher gas flow rate can disrupt the liquid film, causing decreased contact between the gas and liquid phases, further reducing CO2 removal efficiency. In contrast, the rise in CO2 flux as the gas flow rate increases in a descending layer of MEA can be ascribed to heightened turbulence and improved mixing at higher gas flow rates. This augmented turbulence facilitates improved interaction between the gas and liquid phases, resulting in enhanced CO2 transfer from the gas to the liquid. Moreover, the increased gas flow rate leads to a thinner liquid film, reducing film resistance and further boosting the CO2 removal flux57. As a result, while the CO2 removal efficiency declines with higher gas flow rates, as explained earlier, the CO2 flux can increase. This is because the enhanced mass transfer and reduced film resistance associated with increased gas flow rates contribute to a higher rate of CO2 transfer37. In a wet membrane system, membrane thickness and gas permeation velocity may limit the absorption rate. In contrast, a falling film system has a higher surface area-to-volume ratio, resulting in a higher absorption rate58.
Comparison between falling fall and wet mode membranes for the effect of inlet gas flow rate on the CO2 removal efficiency and removal flux.
Based on the simulation results, the falling film membrane system offers a higher CO2 absorption rate and removal flux than the wet membrane system. The falling film system offers an average CO2 removal efficiency of 85% with a removal flux of 0.82 mol/m3 s, while the wet membrane system achieves an average removal efficiency of 60% with a removal flux of 0.55 mol/m3 s. These quantitative findings indicate that the falling film presents a more optimal choice for achieving elevated levels of CO2 removal in specific applications. Good agreement was observed between experimental data and model predictions, with an average error of around 4%. The governing equations of the model have been successfully solved using COMSOL Multiphysics version 6.1. The findings demonstrated that higher gas flow rates could reduce the resistance to mass transfer on the gas side, resulting in an increase in both the removal flux and the reduction of removal efficiency. As the CO2 loading in MEA increases, it can lead to a decrease in both the removal efficiency and removal flux. The strong agreement observed between the mathematical model developed in this study and the experimental data demonstrates the reliability and suitability of the model for investigating the CO2 absorption process in MEA solutions using both wet and falling film membrane systems. Such investigations can expand our understanding of CO2 absorption processes and contribute to the development of more efficient and versatile carbon capture systems.
All data generated or analyzed during this study are included in this published article.
A Correction to this paper has been published: https://doi.org/10.1038/s41598-023-39137-y
Rohani, R., Yusoff, I. I., Amran, N. F. A., Naim, R. & Takriff, M. S. Comparison of separation performance of absorption column and membrane contactor system for biohydrogen upgraded from palm oil mill effluent fermentation. Environ. Prog. Sustain. Energy 40, 25 (2021).
Ghasem, N. CO2 removal from natural gas. In Advances in Carbon Capture 479–501 (Elsevier, 2020).
Olabi, A. G. et al. Membrane-based carbon capture: Recent progress, challenges, and their role in achieving the sustainable development goals. Chemosphere 320, 5 (2023).
Imtiaz, A. et al. A critical review in recent progress of hollow fiber membrane contactors for efficient CO2 separations. Chemosphere 325, 2 (2023).
Ho, C.-D., Chang, H., Chen, Y.-H., Chew, T. L. & Ke, J.-W. Investigation on the performance of CO2 absorption in ceramic hollow-fiber gas/liquid membrane contactors. Membranes (Basel) 13, 25 (2023).
Fattah, I. M. R., Farhan, Z. A., Kontoleon, K. J., Kianfar, E. & Hadrawi, S. K. Hollow fiber membrane contactor based carbon dioxide absorption—stripping: A review. Macromol. Res. 20, 1–27 (2023).
Hou, R., Fong, C., Freeman, B. D., Hill, M. R. & Xie, Z. Current status and advances in membrane technology for carbon capture. Sep. Purif. Technol. 300, 121863 (2022).
Pahija, E., Golshan, S., Blais, B. & Boffito, D. C. Perspectives on the process intensification of CO2 capture and utilization. Chem. Eng. Process. Intensif. 176, 108958 (2022).
Lee, Y., Park, Y.-J., Lee, J. & Bae, T.-H. Recent advances and emerging applications of membrane contactors. Chem. Eng. J. 20, 141948 (2023).
Amirabedi, P. et al. CO2/N2 separation by glycerol aqueous solution in a hollow fiber membrane contactor module: CFD simulation and experimental validation. Sep. Purif. Technol. 10, 42157–42167 (2022).
Wu, J. et al. Characteristics of CO2 absorption by N, N-dimethylethanolamine (DMEA) in hollow fiber membrane contactor. Huagong Jinzhan/Chem. Ind. Eng. Prog. 41, 2132–2139 (2022).
Cesari, L., Castel, C. & Favre, E. Membrane contactors for intensified gas-liquid absorption processes with physical solvents: A critical parametric study. J. Memb. Sci. 635, 119377 (2021).
Noordokht, M. & Abdoli, S. M. The CO2 removal of flue gas using hollow fiber membrane contactor: A comprehensive modeling and new perspectives. Adv. Environ. Technol. 7, 47–56 (2021).
Ghasem, N. Modeling and simulation of CO2 absorption enhancement in hollow-fiber membrane contactors using CNT–water-based nanofluids. J. Membr. Sci. Res. 5, 295–302 (2019).
Ghasem, N. Modeling and simulation of the hollow fiber bore size on the CO2 absorption in membrane contactor. Chem. Prod. Process Model. 15, 25 (2020).
Mousavian, S. et al. Modeling and simulation absorption of CO2using hollow fiber membranes (HFM) with mono-ethanol amine with computational fluid dynamics. J. Environ. Chem. Eng. 8, 25 (2020).
Kianfar, E. & Khazar, S. G. Modeling and computational fluid dynamics (CFD) simulation of CO2 absorption using mono-ethanol amine (mea) Solution in a hollow fiber membrane (HFM) contactor. Comput. Fluid Dyn. Adv. Res. Appl. 20, 97–123 (2021).
Ghobadi, J. et al. Mathematical modeling of CO2 separation using different diameter hollow fiber membranes. Int. J. Greenh. Gas Control 104, 25 (2021).
Ho, C.-D. et al. Theoretical and experimental studies of CO2 absorption in double-unit flat-plate membrane contactors. Membranes (Basel) 12, 25 (2022).
Goyal, N., Suman, S. & Gupta, S. K. Mathematical modeling of CO2 separation from gaseous-mixture using a Hollow-Fiber Membrane Module: Physical mechanism and influence of partial-wetting. J. Memb. Sci. 474, 64–82 (2015).
Yin, Y. et al. Experimental measurement and modeling prediction of mass transfer in a hollow fiber membrane contactor using tertiary amine solutions for CO2 absorption. Ind. Eng. Chem. Res. 61, 9632–9643 (2022).
Fu, H. et al. CO2 capture based on Al2O3 ceramic membrane with hydrophobic modification. J. Eur. Ceram. Soc. 43, 3427–3436 (2023).
Xue, K., Fu, H., Chen, H., Zhang, H. & Gao, D. Investigation of membrane wetting for CO2 capture by gas–liquid contactor based on ceramic membrane. Sep. Purif. Technol. 304, 122309 (2023).
Abdolahi-Mansoorkhani, H. & Seddighi, S. CO2 capture by modified hollow fiber membrane contactor: Numerical study on membrane structure and membrane wettability. Fuel Process. Technol. 209, 25 (2020).
Qi, W., Fu, K., Chen, X., Qiu, M. & Fan, Y. Falling liquid-film on hydrophilic porous ceramic membrane for boosting CO2 absorption. Sep. Purif. Technol. 303, 25 (2022).
Sun, J. et al. Study on robust absorption performance of hydrophilic membrane contactor for direct air capture. Sep. Purif. Technol. 309, 25 (2023).
Qi, W., Fu, K., Chen, X., Qiu, M. & Fan, Y. Falling liquid-film on hydrophilic porous ceramic membrane for boosting CO2 absorption. Sep. Purif. Technol. 303, 122238 (2022).
Zhang, H., Xue, K., Cheng, C., Gao, D. & Chen, H. Study on the performance of CO2 capture from flue gas with ceramic membrane contactor. Sep. Purif. Technol. 265, 118521 (2021).
Kim, S., Scholes, C. A., Heath, D. E. & Kentish, S. E. Gas-liquid membrane contactors for carbon dioxide separation: A review. Chem. Eng. J. 411, 128468 (2021).
Bavarella, S. et al. CO2 absorption into aqueous ammonia using membrane contactors: Role of solvent chemistry and pore size on solids formation for low energy solvent regeneration. Sep. Purif. Technol. 290, 25 (2022).
Bozonc, A.-C., Cormos, A.-M., Dragan, S., Dinca, C. & Cormos, C.-C. Dynamic modeling of CO2 absorption process using hollow-fiber membrane contactor in MEA solution. Energies 15, 24 (2022).
Ghasem, N. Modeling and simulation of the impact of feed gas perturbation on CO2 removal in a polymeric hollow fiber membrane. Polymers 14, 25 (2022).
Elhambakhsh, A. & Keshavarz, P. Sono-hollow fiber membrane contactors: A new approach for CO2 separation by physical/chemical absorbents. J. Nat. Gas Sci. Eng. 101, 25 (2022).
Hidalgo, D., Sanz-Bedate, S., Martín-Marroquín, J. M., Castro, J. & Antolín, G. Selective separation of CH4 and CO2 using membrane contactors. Renew. Energy 150, 935–942 (2020).
Wenten, I. G., Wardani, A. K., Khoiruddin, K. & Ariono, D. Preliminary study of wet-free CO2 absorption through membrane diffuser. IOP Conf. Ser. Mater. Sci. Eng. 823, 25 (2020).
Khan Swati, I. et al. Non-dispersive solvent absorption of post-combustion CO2 in membrane contactors using ionic liquids. J. Mol. Liq. 351, 25 (2022).
Kancherla, R., Nazia, S., Kalyani, S. & Sridhar, S. Modeling and simulation for design and analysis of membrane-based separation processes. Comput. Chem. Eng. 148, 107258 (2021).
Nakhjiri, A. T., Heydarinasab, A., Bakhtiari, O. & Mohammadi, T. Modeling and simulation of CO2 separation from CO2/CH4 gaseous mixture using potassium glycinate, potassium argininate and sodium hydroxide liquid absorbents in the hollow fiber membrane contactor. J. Environ. Chem. Eng. 6, 1500–1511 (2018).
Yuan, C. et al. Modeling of CO2 absorption into 4-diethylamino-2-butanol solution in a membrane contactor under wetting or non-wetting conditions. Carbon Capture Sci. Technol. 5, 24 (2022).
Fu, H. et al. Study on the performance of CO2 capture from flue gas with ceramic and PTFE membrane contactors. Energy 263, 14 (2023).
Li, Y., Ma, Y., Li, Y. & Li, S. Processing and microstructure-permeation properties of silica bonded silicon carbide ceramic membrane. J. Eur. Ceram. Soc. 41, 7525–7532 (2021).
Cussler, E. L. Values of diffusion coefficients. Diffus. Mass Transf. Fluid Syst. 20, 105–145 (1997).
Paul, S., Ghoshal, A. K. & Mandal, B. Removal of CO2 by single and blended aqueous alkanolamine solvents in hollow-fiber membrane contactor: Modeling and simulation. Ind. Eng. Chem. Res. 46, 2576–2588 (2007).
Magnone, E., Lee, H. J., Shin, M. C. & Park, J. H. A performance comparison study of five single and sixteen blended amine absorbents for CO2 capture using ceramic hollow fiber membrane contactors. J. Ind. Eng. Chem. 100, 174–185 (2021).
Iversen, S. B., Bhatia, V. K., Dam-Johansen, K. & Jonsson, G. Characterization of microporous membranes for use in membrane contactors. J. Memb. Sci. 130, 205–217 (1997).
Barth, D., Tondre, C. & Delpuech, J.-J. Stopped-flow investigations of the reaction kinetics of carbon dioxide with some primary and secondary alkanolamines in aqueous solutions. Int. J. Chem. Kinet. 18, 445–457 (1986).
Rezaeian Kouchi, L., Mohammad Ghoreishi, S. & Reza Rahimpour, M. Simulation study of carbon dioxide capture in a novel rotating liquid sheet contactor. Fuel 333, 126322 (2023).
He, X., Lei, L. & Chu, Y. In Chapter 9—Facilitated Transport Membranes for CO2 Removal From Natural Gas (eds Basile, A. & Favvas, E. P.) 261–288 (Elsevier, 2018). https://doi.org/10.1016/B978-0-12-813645-4.00009-X.
Basile, A., Gugliuzza, A., Iulianelli, A. & Morrone, P. 5—Membrane technology for carbon dioxide (CO2) capture in power plants. In Woodhead Publishing Series in Energy (eds Basile, A. & Nunes, S. P.) 113–159 (Woodhead Publishing, 2011). https://doi.org/10.1533/9780857093790.2.113.
Lei, L. et al. Carbon membranes for CO2 removal: Status and perspectives from materials to processes. Chem. Eng. J. 401, 126084 (2020).
Demontigny, D., Tontiwachwuthikul, P. & Chakma, A. Parametric studies of carbon dioxide absorption into highly concentrated monoethanolamine solutions. Can. J. Chem. Eng. 79, 137–142 (2001).
Hernández-Romero, I. M., Flores-Tlacuahuac, A., Nápoles-Rivera, F., Esquivel-Patiño, G. G. & García-Pardo, M. L. Multi objective optimization of the amines- CO2 capture absorption-desorption process by a non-equilibrium rate model. Chem. Eng. Res. Des. 187, 93–104 (2022).
Ma, C., Pietrucci, F. & Andreoni, W. Capture and release of CO2 in monoethanolamine aqueous solutions: New insights from first-principles reaction dynamics. J. Chem. Theory Comput. 11, 3189–3198 (2015).
Melnikov, S. M. & Stein, M. The effect of CO2 loading on alkanolamine absorbents in aqueous solutions. Phys. Chem. Chem. Phys. 21, 18386–18392 (2019).
Putta, K. R., Pinto, D. D. D., Svendsen, H. F. & Knuutila, H. K. CO2 absorption into loaded aqueous MEA solutions: Kinetics assessment using penetration theory. Int. J. Greenh. Gas Control 53, 338–353 (2016).
Aghel, B., Janati, S., Wongwises, S. & Shadloo, M. S. Review on CO2 capture by blended amine solutions. Int. J. Greenh. Gas Control 119, 103715 (2022).
Zhang, F., Guo, L., Ding, Y., Zhu, X. & Liao, Q. Flow pattern and CO 2 absorption in a falling film reactor with mixed aqueous solution of ionic liquid and MEA. Appl. Therm. Eng. 138, 14 (2018).
Sehgal, S., Alvarado, J. L., Hassan, I. G. & Kadam, S. T. A comprehensive review of recent developments in falling-film, spray, bubble and microchannel absorbers for absorption systems. Renew. Sustain. Energy Rev. 142, 110807 (2021).
This work was supported by the United Arab Emirates University research office, UAE (Grant No. 31N374).
Department of Chemical and Petroleum Engineering, United Arab Emirates University, PO Box 15551, Al Ain, UAE
You can also search for this author in PubMed Google Scholar
The entire article is modeled and simulated by the corresponding author.
The author declares no competing interests.
Springer Nature remains neutral with regard to jurisdictional claims in published maps and institutional affiliations.
The original online version of this Article was revised: The original version of this Article contained an error in Reference 14, which was incorrectly given as: Ghasem, N. & Al-Marzouqi, M. Modeling and experimental study of carbon dioxide absorption in a flat sheet membrane contactor. J. Membr. Sci. Res. 3, 25 (2017). The correct reference is: Ghasem, N. Modeling and Simulation of CO2 Absorption Enhancement in Hollow-Fiber Membrane Contactors using CNT–Water-Based Nanofluids. J. Membr. Sci. Res. 5, 295–302 (2019).
Open Access This article is licensed under a Creative Commons Attribution 4.0 International License, which permits use, sharing, adaptation, distribution and reproduction in any medium or format, as long as you give appropriate credit to the original author(s) and the source, provide a link to the Creative Commons licence, and indicate if changes were made. The images or other third party material in this article are included in the article's Creative Commons licence, unless indicated otherwise in a credit line to the material. If material is not included in the article's Creative Commons licence and your intended use is not permitted by statutory regulation or exceeds the permitted use, you will need to obtain permission directly from the copyright holder. To view a copy of this licence, visit http://creativecommons.org/licenses/by/4.0/.
Ghasem, N. Efficient CO2 absorption through wet and falling film membrane contactors: insights from modeling and simulation. Sci Rep 13, 10994 (2023). https://doi.org/10.1038/s41598-023-38249-9
DOI: https://doi.org/10.1038/s41598-023-38249-9
Anyone you share the following link with will be able to read this content:
Sorry, a shareable link is not currently available for this article.
Provided by the Springer Nature SharedIt content-sharing initiative
By submitting a comment you agree to abide by our Terms and Community Guidelines. If you find something abusive or that does not comply with our terms or guidelines please flag it as inappropriate.
Scientific Reports (Sci Rep) ISSN 2045-2322 (online)
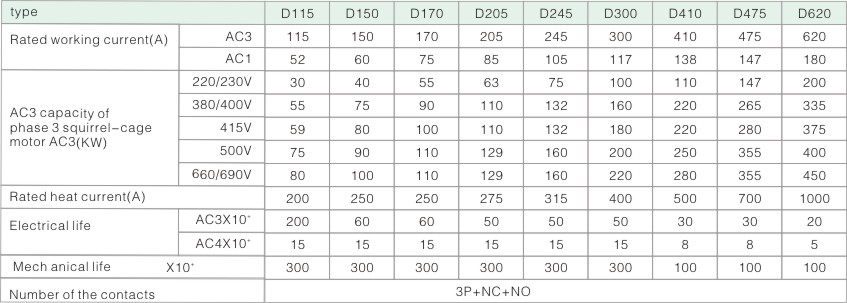
Ac Plug Socket Sign up for the Nature Briefing newsletter — what matters in science, free to your inbox daily.