Thank you for visiting nature.com. You are using a browser version with limited support for CSS. To obtain the best experience, we recommend you use a more up to date browser (or turn off compatibility mode in Internet Explorer). In the meantime, to ensure continued support, we are displaying the site without styles and JavaScript.
Scientific Data volume 11, Article number: 190 (2024 ) Cite this article Wave Spectrum Gauge
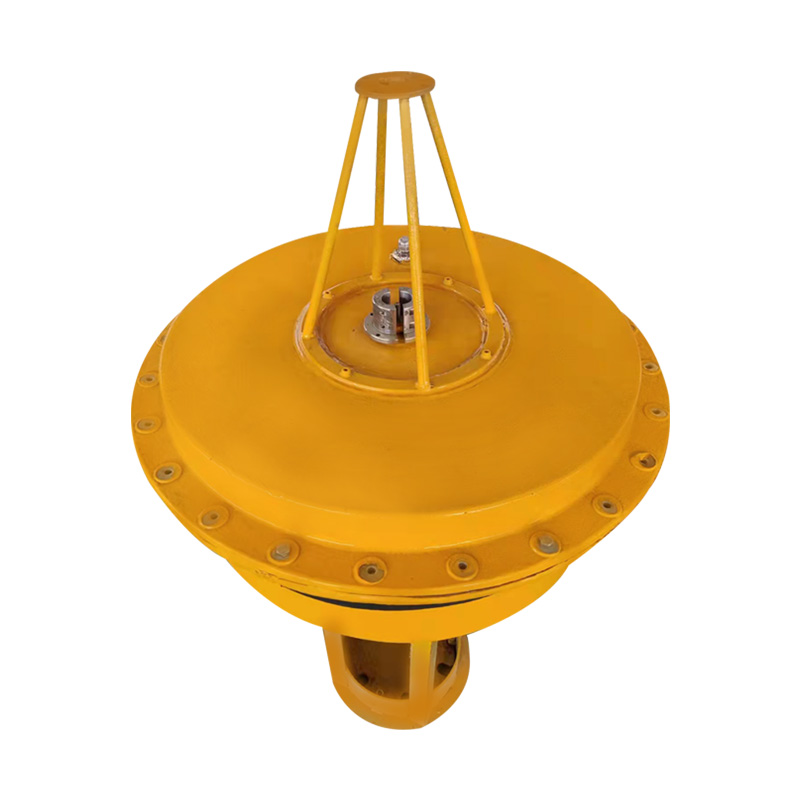
Wind wave observations in shallow coastal waters are essential for calibrating, validating, and improving numerical wave models to predict sediment transport, shoreline change, and coastal hazards such as beach erosion and oceanic inundation. Although ocean buoys and satellites provide near-global coverage of deep-water wave conditions, shallow-water wave observations remain sparse and often inaccessible. Nearshore wave conditions may vary considerably alongshore due to coastline orientation and shape, bathymetry and islands. We present a growing dataset of in-situ wave buoy observations from shallow waters (<35 m) in southeast Australia that comprises over 7,000 days of measurements at 20 locations. The moored buoys measured wave conditions continuously for several months to multiple years, capturing ambient and storm conditions in diverse settings, including coastal hazard risk sites. The dataset includes tabulated time series of spectral and time-domain parameters describing wave height, period and direction at half-hourly temporal resolution. Buoy displacement and wave spectra data are also available for advanced applications. Summary plots and tables describing wave conditions measured at each location are provided.
Reliable measurements of nearshore wave conditions are essential for calibrating, validating and improving shallow-water wave transformation models1,2, which are used by coastal scientists and engineers to define local wave climates and extremes, drive shoreline response and coastal evolution models, and investigate and predict coastal processes, dynamics and hazards3,4,5. The local wave conditions impacting a beach or rocky shore may vary considerably from adjacent coasts for the same offshore wave conditions1, due to alongshore variation in coastline orientation, the presence of coastal islands, shoreline shape, and seabed bathymetry and texture across the wave shoaling zone (shoreface). Ocean wave buoy networks6, satellite altimeters7, and deep-water wave models8 (some with data assimilation) provide historical and forecast ocean wave data at a global scale, however, shallow-water wave observations are rarely available. This is a critical knowledge gap for wind-wave9 and coastal geoscience and engineering10 research in Australia.
Cost, resource, logistical and time limitations mean that it is often infeasible to deploy a wave measurement device (e.g., wave buoy) to study local wave conditions at sites of interest for coastal research or management. A shallow-water wave model is usually applied to simulate nearshore wave conditions based on measured or modelled deep-water wave data1,2. The limited availability of nearshore wave observations means that shallow-water wave models may be subject to limited or no calibration and validation in practice, despite having crucial importance in simulating coastal processes and hazards of interest3,4,5. This is most problematic in settings with complex seabed geomorphology, where modelled wave conditions may be more sensitive to the design (including bathymetry and bed friction) of shallow-water wave models. Without nearshore wave observations covering different shore (sandy, gravel, rocky) and seabed (sediment, reef, mixed) types, shoreface slopes, and ocean wave exposure, the accuracy of shallow-water wave modelling in practice often remains poorly quantified.
We present a growing dataset of in-situ shallow-water (<35 m depth) wave observations along the coast of New South Wales (NSW), southeastern Australia (Fig. 1). The ongoing program of short-term (months to years) wave buoy deployments covers a range of coastal settings and geomorphology, including those where infrastructure and properties are known to be at risk from coastal erosion and wave inundation11,12. Since March 2016, 81 moored wave buoy deployments have been carried out at 20 locations (Fig. 1), collecting over 7,000 days of half-hourly wave data as of 30 November 2023 (Tables 1–3). The dataset captures both fair-weather and storm-wave conditions (significant wave height, Hm0 > 3 m), including a storm in June 201612 during which one wave buoy measured Hm0 approaching 7 m, and multiple Hm0 observations exceeding 5 m at other locations to date (Tables 1, 2). We provide commonly used time series data, including spectral and time-domain parameters for wave height, period and direction at half-hourly temporal resolution, with plots and tables summarising measured wave conditions at each location. Parameter data from active wave buoy deployments can also be viewed and downloaded in real time. For advanced applications, wave buoy displacement data and wave spectra data are also made available through the Australian National Wave Archive (see Data Records).
The 20 nearshore wave buoy deployments along the coast of New South Wales, Australia (a,b) that were completed or in progress at 31/8/2023 (Tables 1–3). Locations of seven long-term offshore wave buoy deployments13 (65–80 m water depths) are also shown. Site location maps (c–m) showing aerial imagery, high-resolution lidar topography-bathymetry14 and seabed landforms15 mapping, with general bathymetry, are provided for 18 locations for which final data is available and included in the dataset (Tables 1–2). All site maps are oriented north-up with 1-km scale bars (white) and the names of deployments shown in each panel are listed from north to south at top left.
The nearshore wave data will be of interest to wind-wave and coastal researchers in Australia and abroad, providing rare observation data for developing and evaluating shallow-water wave models for local or global applications. Data from seven long-term deep-water wave buoys spanning the study area (Fig. 1) are available separately13 and provide regional wave climate information and ocean wave boundary conditions for modelling. High-resolution (5 m grid) seamless coastal topography-bathymetry data14 and seabed landforms (sediment, rocky reef) mapping15,16 are also available separately from state-wide airborne lidar surveys reaching 30 m water depth on average17 (Fig. 1), and in deeper water where multibeam echosounder surveys have been completed16,17 (see Usage Notes). The wave buoy data will also interest researchers and applied engineers studying coastal processes, dynamics and hazards. Nearshore wave buoy deployments adjacent to multi-decadal coastal monitoring sites at Moruya (Bengello) Beach18 and Collaroy-Narrabeen Beach19 (Fig. 1) are ongoing, and together, provide community testbeds for international coastal modelling studies20,21,22.
Our motivations are to improve the understanding and prediction of coastal wave conditions in southeast Australia, to provide nearshore wave data for coastal processes research and hazard management studies23,24, and to advance shallow-water wave and coastal modelling methods globally through the provision of essential observation data. The nearshore wave buoy data are also being used to improve a regional-scale high-resolution coastal wave model covering the NSW coast25,26. Local-scale wave data are increasingly needed in NSW and globally as community exposure to coastal hazards increases with both development and climate change pressures11,20. Nearshore wave buoy data collected in our program have already been applied in coastal processes research27 and coastal risk management studies28, and to develop early warning systems for rocky shore wave hazards29 and storm impacts on sandy beaches30.
The NSW coast is microtidal, wave-dominated and is situated on a passive tectonic continental margin that is unusually steep and narrow by global comparison31, resulting in low attenuation of wave energy across the mid to outer continental shelf32. The coastline trends NNE to SSW (Fig. 1) with regional coastline orientation controlled by the geological framework of the margin and local variations by embayment geometry and sediment availability31. Coastal embayments are generally broader and shallower in the north and narrower and deeper in the south and contain sandy bay-barrier beaches extending between sections of coastal cliffs and rocky headlands33. Just over half of the 2,000 km ocean coastline is sandy beaches, with nearshore wave conditions influencing the orientation of sandy shorelines31.
The ocean wave climate is moderate-high energy by global standards, with long-term deep-water wave buoy records indicating mean significant wave height and period of 1.6 m and 8 s in the central NSW region34. The wave climate has a mild seasonal bias, being more energetic in austral winter months than austral summer, and experiences inter-annual to inter-decadal variability associated with climate cycles34,35. The directional wave climate varies along the coast, with the north experiencing more easterly wave directions compared to the south36. Storm wave conditions are influenced by a range of synoptic systems that are characterised by their origins and behaviour37,38, and deep-water significant wave heights up to 9 m and maximum wave heights over 18 m have been recorded by a network of seven long-term offshore wave buoy deployments13 (Fig. 1). A significant wave height threshold of 3 m is typically used to distinguish ‘storm’ from ‘fair-weather’ wave conditions37.
We have made 81 temporary moored wave buoy deployments at 20 nearshore locations in water depths <35 m along the NSW coastline (Fig. 1), from March 2016 to November 2023, and data collection is ongoing. Tables 1–3 provide details of the deployment positions, durations, data records and instruments used at each nearshore location, ordered from north to south for comparison with Fig. 1. The locations have been chosen based on four criteria: (1) sample a variety of coastal settings and bathymetric complexity; (2) capture concurrent data in nearby locations with contrasting wave exposure; (3) collect data at locations with known coastal hazard risks and/or active coastal process studies; and (4), complement other field operations such as multibeam echosounder seabed mapping17.
We collect nearshore wave data using small translational wave buoys deployed on temporary moorings in shallow coastal waters (Fig. 2). Deployments are placed either outside the surf zone near the base of the upper shoreface (e.g., 12 m water depth) or around the lower shoreface-inner continental shelf transition (e.g., 30 m water depth). The duration of buoy deployments varies depending on data requirements and operational considerations. For example, a brief 5-week deployment at Gerroa supported a coastal process study there27, while 12-month and 16-month deployments at Figure Eight Pools and Stockton Beach were intended to establish the relationship between offshore and nearshore wave conditions for hazard investigations28,29 (Tables 1, 2). The longest deployments are adjacent to multi-decadal beach survey datasets at Moruya (Bengello) Beach18 and Collaroy-Narrabeen Beach19 and are ongoing. Deployments are visited for servicing at regular intervals (e.g., 5 weeks for Datawell buoys and 4-6 months for Spotter buoys) to remove biofouling or replace the wave buoy and mooring with fresh equipment, and to retrieve onboard recorded data. The number of service deployments at each location is shown in Tables 1–3, and details of service deployments are provided in the readme file of each deployment data package. See Technical Validation for further details on erroneous data, data loss and omission.
(a) Spotter buoy on a paired-float mooring at Bengello; (b) Spotter buoy on a high-visibility mooring at Deeban; (c) heavy fouling of a Datawell buoy and mooring at Boomerang B with goose barnacles (Lepas pectinata) and green algae (Ulva sp.); (d) heavy fouling of a Spotter buoy and mooring at Merimbula with blue mussels (Mytilus galloprovincialis). See Fig. 1 for deployment locations.
Two types of directional GPS/GNSS (hereafter GNSS for brevity) wave buoys have been used in the program: Datawell Waverider DWR-G439 (Table 1) and Sofar Ocean Spotter40 (Tables 2, 3). Both instruments are small-format buoys that measure the wave field by recording their horizontal and vertical translation (displacement) using a satellite positioning receiver, rather than by measuring buoy motion and orientation physically using onboard mechanical instruments, such as accelerometers, gyros and compass. Key differences between the Datawell and Spotter buoys include the shape, fabrication materials and weight of their hulls, power systems, data storage and transmission, satellite positioning networks, and the frequency at which they record their displacement.
The Datawell buoy39 has a spherical steel hull of 0.40 m diameter (0.46 m with rubber fender) and weighs 17 kg as deployed with batteries and ballast chain. It operates on a battery power system with a battery life of around 30 days and thus require service visits every 4–5 weeks to maintain a continuous data record. The satellite receiver unit measures displacement using the Doppler shift of the satellite data signal. Displacement data are recorded continuously at 1.28 Hz, resulting in 2304 displacement records per half-hour sampling period, which are stored on a compact flash data card41. Real-time data transmission on our DWR-G4 units was limited to HF radio, which was rarely used to conserve batteries.
The Spotter buoy40 has a pentagonal upper/spherical lower plastic hull of 0.42 m diameter and weighs 7.6 kg as deployed with ballast chain. Operating on a solar-battery power system with five 2-W solar panels the Spotter buoy can be deployed indefinitely, although biofouling limits deployment duration in practice. The satellite receiver measures displacement using the Doppler shift and position data and records its displacement at 2.5 Hz, resulting in 4500 records per half-hour sampling period, which are stored on an SD data card. Spotter buoy versions 1 and 2 featured Iridium satellite telemetry of half-hourly onboard-computed wave spectra (coefficients) or a limited set of bulk wave parameters42 (describing wave height, period and direction), wind speed (inferred from measured wave spectra43) and buoy position. A water surface temperature sensor was added in version 2 and a cellular network data telemetry option in version 3.
To evaluate consistency in wave measurement between the Datawell and Spotter buoys we conducted a 2-month paired deployment at Old Bar (Fig. 1) during October-December 2018, where Datawell and Spotter buoys were deployed 100 m apart in similar water depths and on identical moorings. As both instruments measure displacement using the GNSS method, it was anticipated that the wave data measured by each should be comparable, although variations might emerge from different hull designs, satellite receiver units and firmware/software. The different displacement recording frequencies might also influence the derived wave data. The buoy comparison experiment found that wave data from the two instruments is comparable for our purposes, the key difference being in the occurrence and nature of data artefacts arising from satellite position observations. See the Technical Validation section for results of the Datawell-Spotter buoy comparison and further details on erroneous data from satellite position observations.
Deployments from March 2016 to December 2018 were made using a pool of four Datawell DWR-G4 buoys: two from NSW Environment and Heritage (NSWENV), one from the Sydney Institute of Marine Science (SIMS) through support from the Integrated Marine Observing System (IMOS), and one from the Water Research Laboratory (UNSW Sydney). Two Spotter-v1 buoys were purchased through SIMS-IMOS on behalf of NSWENV in June 2018. An additional four Spotter-v1 buoys were purchased by NSWENV in June 2019, three Spotter-v2 buoys in June 2021 and one Spotter-v3 buoy in April 2023. The Water Research Laboratory purchased a Spotter-v1 buoy in September 2019 to support ongoing deployments at Collaroy-Narrabeen Beach (Fig. 1). Following the Datawell-Spotter buoy comparison at Old Bar, the Datawell deployments (Table 1) at Farquhar and Old Bar (Fig. 1) were replaced with Spotter buoys (Table 2) during a service visit on 4 December 2018. Subsequently, Datawell buoys were only used at the Boomerang B deployment (Fig. 1), which was replaced with a Spotter buoy on 24 July 2019 (Table 2). The transition to Spotter buoys reduced the logistical requirements of maintaining deployments by increasing the duration between service visits, allowing for longer deployments and more simultaneous locations.
Our standard temporary mooring design follows Datawell’s recommendations for the DWR-G4 buoy in shallow water (p. 95)41 with some minor variations. They comprise a medium-size sand bottom boat anchor with at least 10 m of 8–10 mm galvanised steel chain to keep the mooring in place, and weighted Dyneema line connecting the anchor chain to the mooring floats and wave buoy at the water surface. This includes a length of line about twice the deployment water depth between the anchor chain and surface floats (e.g., 20–25 m of line for deployments around 12 m water depth). An additional 30 m length of unweighted line is added between the anchor chain and the weighted upper mooring line for deployments in 30 m water depth. Our weighted lines feature 3–4 elongate fishing sinkers (170 g) spliced and taped into the line about 1 m apart along the central section of line.
The surface floats for our standard mooring design include a pair of 0.25 m diameter spherical centre bore floats with 8.5 kg buoyancy rating each (Fig. 2a). In settings where increased mooring visibility is required (e.g., high vessel traffic), a 0.6 m base diameter yellow special navigation mark float (30 kg buoyancy rating) with a marine lantern is used with high-visibility surface line floats (Fig. 2b). The surface floats reduce the influence of the mooring on the movement of the wave buoy by maintaining slack line near the water surface between the floats and wave buoy. They also increase the visibility of the mooring to assist with navigation safety and reduce the chance of vessel strike. Both Datawell and Spotter wave buoys are equipped with yellow flashing navigation marker lights for night-time visibility.
For the standard mooring with paired surface floats (Fig. 2a), the wave buoy (with supplied ballast chain) is connected to the floats by 10–15 m of weighted line. For a high-visibility navigation mark mooring (Fig. 2b) the central sector of the weighted buoy line is replaced with a pair of 0.48 m length marker floats (9 kg buoyancy rating each) that are connected with 8 mm chain zip-tied along the float line grooves (Fig. 2b). Under the influence of changing currents or wind, the wave buoys can move freely within an arc (i.e., watch circle) around the mooring anchor point, while the surface floats absorb the motion of the mooring and reduce any influence on wave buoy displacement.
Moorings and buoys are susceptible to fouling by sessile marine fauna in the shallow coastal waters where our deployments are located, which left unchecked adds considerable weight to the mooring and reduces the efficacy of the surface floats in reducing mooring influence on the buoy. We have found that common marine fouling organisms in our settings include green “sea lettuce” algae (Ulva sp.), goose barnacles (Lepas pectinata), acorn barnacles (Chthamalus antennatus), sea squirts and sea tulips (Pyura sp.), and blue mussels (Mytilus galloprovincialis). Mooring entanglement on mobile marine fauna (e.g., whales and turtles) has not occurred with our buoy deployments to date, however, we are testing fauna-friendly mooring designs comprising flexible tubing sections around mooring lines to reduce any potential risk of marine fauna entanglement.
Datawell buoy deployments were visited every 4–5 weeks to change batteries and retrieve data, during which the buoy and mooring were cleaned onboard the vessel and redeployed, with the buoy and mooring replaced with fresh equipment every third visit. Spotter buoy deployments are visited every 4-6 months on average with both the buoy and mooring replaced with fresh equipment during each service visit. The dates of buoy deployments, service visits and buoy retrievals are provided in the readme metadata files with each data package. Heavy fouling that has been observed, upon visiting, to compromise wave buoy behaviour has only occurred when service visits were significantly delayed due to operational limitations (Fig. 2c,d). In such cases, data records have been inspected and truncated if they were deemed to be compromised – see Technical Validation (Data loss and omission).
Deployment, service and retrieval operations have been primarily carried out using our research vessels, RV Bombora (11.8 m fibreglass Stebercraft) and Badoo (6.4 m aluminium Seatamer). Deployment locations are recorded using RTK-GNSS survey or vessel navigation systems to ensure that moorings are redeployed at the same position during each service visit. The wave buoy and mooring lines are deployed first, and the chain and anchor are deployed last at the deployment location. Datawell buoys are switched on by connecting the power cable to the electronics unit several minutes prior to deployment. Spotter buoys are switched to Run (recording) mode moments prior to deployment and switched to Stand-by mode moments after retrieval. Further description of our deployment methods can be found within the Australian Wave Buoy Operations and Data Management Guidelines Manual44 available from Ocean Best Practices.
Our data processing methods follow long-established techniques used for spectral and time-domain analysis of surface buoy displacement measurements45,46,47. We apply our methods uniformly to the displacement data retrieved from onboard data cards in binary (Datawell) or ascii (Spotter) formats rather than using proprietary data processing tools. The key difference in the displacement data records between Datawell and Spotter buoys is that, due to the different sampling frequencies, in each half hour there are 2304 Datawell displacement records and 4500 Spotter displacement records. Both buoys commence measuring shortly (minutes) after they are powered-up (Datawell) or switched to Run mode (Spotter). By noting those times, and the times that they are deployed and retrieved from the water, we discard the beginning and end of the buoy displacement records and segment the data into regular half-hour packages beginning from the first UTC half hour passed following deployment.
We filter the raw displacement data to address known artefacts that affect GNSS wave buoy displacement measurements, which are caused by interruptions in the satellite data stream. The artefacts appear as erroneous spikes or sawtooth patterns in heave (z) displacements, in particular, which introduce errors in derived wave spectra and parameters48,49,50,51. Following an approach described by Andrews & Peach51, we apply a coarse spike filter to each half hour of displacement data to remove outliers exceeding five times the standard deviation and to improve the performance of high-pass filtering. We then apply a second-order Butterworth high-pass filter to remove any signals in the x, y and z displacement data that are beyond the recording frequencies of the buoys, using a cut-off of 0.029 Hz (Spotter buoy sampling limit). All subsequent processing is carried out using the filtered displacement set for each half-hour sampling period. Displacement data artefacts are further discussed in Technical Validation (Erroneous data).
For spectral analysis, the derivation of variance density spectra, spectral moments and bulk parameters follow similar standard approaches that are used by Datawell41 and Sofar Ocean42 to calculate onboard spectral and parametric data. Each continuous half-hour displacement record is divided into non-overlapping consecutive data segments (n = 256), from which consecutive spectra are derived and then averaged. Due to the varying sampling frequencies, each consecutive displacement data segment and derived spectra equates to sampling periods of 200 s (Datawell) and 102.4 s (Spotter). The wave spectra for each half hour from which bulk parameters are derived is therefore determined by averaging 9 (Datawell) or 17 (Spotter) consecutive spectra. The frequency resolution of the spectra also varies due to sampling rate, being 0.005 Hz for Datawell buoys41 and ≈ 0.0097 Hz for Spotter buoys42.
We use the fast Fourier-transform (FFT) method to derive surface elevation, x-displacement and y-displacement variance density spectra for each data segment, from which the x-y co-spectrum, x-z quad-spectrum and y-z quad-spectrum are constructed. Bulk parameters describing wave height and period for each half-hour displacement data record are calculated from the frequency moments of the variance density spectra as described in Table 4. Directional properties are estimated from the lowest-order directional moments using the co/quad-spectra and displacement spectra45,46. Directions associated with the mean and peak frequencies are calculated as described in Table 4.
We use standard zero-upcrossing analysis methods47 to calculate time-domain wave height and period parameters using the same regular half-hour buoy displacement data packages that are used for spectral processing. Time-domain parameters are computed for comparison with corresponding spectral parameters and to provide a comprehensive and flexible suite of parameters to support most applications. The time-domain parameters are described in Table 4. The time series data table of processed spectral and time-domain wave parameter data is constructed by appending the parameter values calculated from each consecutive half-hour buoy displacement record.
A key difference between the processed data from completed deployments and the data computed onboard and transmitted from Spotter buoys in real time (which is provided for active deployments, see Data Records) is that our processed data are derived from half-hour displacement data packages commencing on the hour and half hour. In contrast, real-time data that is computed onboard and transmitted from Spotter buoys derive from half-hour displacement data segments that commence from whatever time the buoy was switched to Run mode and began sampling. This is important for using the nearshore wave buoy data with concurrent data from wave hindcast/forecast models (in regular UTC time) or the offshore wave buoy network along the NSW coast13 (Fig. 1). The offshore buoys are large-format (0.9 m hull diameter) Datawell Waverider buoys that synchronise data capture and processing to regular UTC hours, with both real-time and post-processed data provided in Australian Eastern Standard Time (AEST)13. Following Datawell41 and Sofar Ocean42 conventions for onboard processed buoy data, our wave parameter time-series data are time-stamped with the end time (AEST) of each half-hour displacement dataset from which the parameters were derived.
Our quality control objectives are to preserve the maximum amount of data measured by the wave buoys, while identifying for the end user, any data that may be compromised or less rigorous and which therefore should be interpreted and used with caution. We follow published standards for oceanographic data quality control and flagging52,53,54 with some modifications and extensions as described below. In our data records, we prioritise post-processed wave parameter data derived following our methods above (see Data processing), but also provide onboard computed wave parameter data for any timestamps where buoy displacement data were not recovered.
Four data quality fields (Qflag, Qcode, Percent, DoF; Table 4) are included in our parameter time-series data tables to describe the rigour and perceived quality of each data point.
The Percent field shows the proportion of each half-hour period that displacement data were recorded by the buoy. A value of 100 indicates that a full half-hour set of displacement data (e.g., 2304 records for a Datawell, 4500 records for a Spotter) was recorded and used in the data processing. Values between 0 and 100% indicate partial loss of displacement data, which may occur due to satellite receiver obstruction, data card write issues or during a mooring service when there is no instrument in the water. A Percent value of zero indicates that no displacement data were recorded during that half hour sampling period. The DoF field provides the corresponding degrees of freedom for the statistical calculation of wave spectra from which spectral parameters are derived. Due to different sampling rates, the degrees of freedom corresponding to a complete displacement data record (Percent = 100) are 36 for a Datawell buoy and 68 for a Spotter buoy. See Technical Validation (Data loss and omission) for more information on data completeness.
The Qflag field provides a primary level quality flag consistent with published standards52,53,54 that identifies each data point as pass (1), suspect (3), fail (4) or absent (9) based on the results of diagnostic testing. Users wishing to exclude suspect, erroneous or absent data points from their analysis or applications can use the Qflag field to sort, extract or omit data without further interrogation. Of the deployment data summarised in Tables 1, 2, over 97% of half-hourly data points were evaluated as pass by diagnostic tests (Table 5).
Suspect data (Qflag 3) are identified using range and rate of change tests53, with a data point flagged as suspect if any parameter value violates the test condition for that parameter. Range tests flag parameter values outside of acceptable ranges as follows: Hm0 (0.1–10 m), Tp (3–21 s), σp (0.07–80°) and temperature (5–55°). Rate of change tests flag change in parameter values between successive half-hour timesteps that exceed acceptable limits as follows: Hm0 (2 m), Tp (10 s), θp (50°), σp (25°) and temperature (2°).
Anomalous data that are likely compromised by displacement measurement errors (Qflag 4) are identified using a mean and standard deviation filter53, which is applied to Hm0 and Tm02 (Tm01 for real-time Spotter data as Tm02 is not provided) using a 6 hour (n = 12) moving window and 2-standard deviation filter. A data point is flagged as fail (Qflag 4) if both the wave height and period parameter values within the moving window exceed their respective filter values. All data points where Tp > 21 s are also flagged as fail. The tests capture wave parameter values derived from erroneous spectra that arise due to displacement data artefacts from satellite receiver errors48,49,50,51. See Technical Validation (Erroneous data) for further details.
The Qcode field provides a secondary level quality code that summarises the provenance, completeness and quality of the data provided for each half hour period. Table 5 lists all potential Qcode values, including their relation to Qflag values, and describes the nature of the displacement data recorded by the buoy and the wave parameter data provided in the time series table for each case. The rate of occurrence of each Qcode value is also provided.
Data with Qcode values 1–3 passed diagnostic tests, however, for 2 an incomplete but sufficient buoy displacement data record was available for data processing, while for 3 an absent or insufficient (Percent < 23) displacement data record was present and onboard computed (real-time) wave parameter data are provided instead. The threshold of 23% displacement data retention for each half-hour timestamp, which is the minimum used to calculate parameters from displacement data stored on the buoy data card, equates to a minimum of 4 Spotter spectra for spectral averaging (DoF = 16). The Datawell buoys either store a complete displacement record or no displacement data for each half-hour sampling period. When Percent < 23 (including Percent = 0), wave parameter values (if available) processed onboard Spotter buoys for the irregular timestamp falling within the regular half-hour sampling period are included in the parameter time series data instead (Qcode 3, 5 and 8, Table 5). This is because in some cases, parameter data may have been computed and transmitted by the buoy within a half-hour sampling period during which few or no displacement data were recorded to the data card. A Qflag value of 3 (suspect) is applied when data pass diagnostic tests but Percent < 100.
A Qcode value of 4 or 5 indicates that some parameters at that timestamp were flagged as suspect by diagnostic tests (Qflag 3), whether data provided were calculated from buoy displacements following our methods (Qcode 4), or onboard computed parameters have been provided (Qcode 5). Qcode values 6, 7 and 8 indicate that some parameters at that timestamp failed diagnostic tests (Qflag 4), and the parameters provided were calculated from either a complete (Qcode 6) or incomplete (Qcode 7) displacement record, or onboard computed parameters have been provided (Qcode 8). Qcode value 9 always occurs with Qflag value 9, following standard conventions for identifying missing data52,53,54, and indicates that neither displacements nor real-time data were available for that timestamp (e.g., buoy failure or a mooring service visit).
All half-hour timestamps from buoy deployment to retrieval are included in the time series data tables regardless of missing data points (see Data loss and omission), which can be readily identified by the Qflag/Qcode values. An exception is if the data record has been truncated at the end of a deployment to omit data that were either deemed to be compromised by mooring influence from biofouling or were absent due to buoy failure. The number of occurrences of each Qflag/Qcode combination in each completed deployment data record is provided in the readme file included with each data package, and the proportion of each Qcode in the dataset (all deployments) to date are provided in Table 5. It is at the user’s discretion to include or exclude data in their application based on their needs and appraisal of data quality.
Readers are directed to Technical Validation for further details on: Offshore-nearshore buoy comparison, Datawell-Spotter buoy comparison, Erroneous data, and Data loss and omission.
A static version of the wave buoy displacement data, spectral data and wave parameter time series data in netCDF format, for all completed deployments at the time of publication (Tables 1, 2), is available from the Australian Ocean Data Network55 to accompany this data descriptor. The static dataset will not be updated as underway (Table 3) and future wave buoy deployments are completed.
Many data users will be interested in accessing the wave parameter data in CSV format. The NSW Sharing and Enabling Environmental Data (SEED) portal56 hosts an up-to-date version of the wave parameter time series data (completed deployments)57 in CSV format within zip packages that also contain data summary plots and tables (described below). The SEED portal also includes an online viewer for real-time wave parameter time series data (active deployments)58 from underway wave buoy deployments. New data packages will be added to the SEED portal upon the completion of underway and future wave buoy deployments.
For advanced data users, up-to-date versions of the wave buoy displacement data, spectral data and wave parameter time series data in netCDF format are available from the Australian National Wave Archive dataset on the Australian Ocean Data Network59. The netCDF data formats included in the static and National Wave Archive datasets are described further below.
Readers are directed to the Usage Notes for details on complementary data that may be useful for data applications, including high-resolution coastal bathymetry and landforms data covering the entire NSW coastline and offshore (deep-water) wave buoy data (Fig. 1).
The nearshore wave buoy datasets described here contribute to the Australian Research Data Commons (ARDC) project, Catching Oz Waves: National infrastructure for in-situ wave observations60.
Data packages as described here for completed wave buoy deployments can be downloaded from the SEED portal dataset page57 under Dataset Packages.
Each deployment data package comprises one zip folder containing the parameter time series data table and wave height-direction exceedance tables in CSV format, summary plots of the deployment data, and a readme file containing metadata about the entire deployment and individual service deployments. The naming format for the data packages is as follows and is also included as a prefix for all files within each data package:
StartDate: start of deployment date in YYYYMMDD
EndDate: end of deployment date in YYYYMMDD
WaterDepth: water depth below sea level (Australian Height Datum - AHD) to the nearest metre (m)
Location: name of deployment location (Tables 1–3)
Three data tables (CSV format) are included in each deployment data package:
A parameter time series data table [Parameters] containing a set of widely used spectral and time-domain wave parameters with metadata and data quality fields (Table 4). Data are provided at half-hour temporal resolution for the duration of each deployment and are time-stamped (AEST) with the end time of the half-hour buoy displacement record from which they were derived.
Two wave height-direction exceedance tables based on the mean (θm) [ExceedanceDm] and peak (θp) [ExceedanceDp] wave directions as summarised in the provided wave rose plots (see below). Fail data points (Table 5) are omitted from the exceedance table calculations.
Data plots (PNG format) are included with each deployment data package to allow users to quickly inspect and interpret the data measured during each deployment:
A summary time-series plot [Summary] showing key wave height (Hm0, Hmax), period (Tm02, Tp,) and direction (θm, θp) parameters over the duration of each deployment, which also identifies data points that were flagged as suspect or fail by diagnostic tests (Fig. 3).
Example time series parameter data summary plot from Spotter buoy deployments at Old Bar B (Fig. 1, Table 2) showing measured wave height (a), period (b) and direction (c) parameters. Marker symbols on the mean parameter (Hm0, Tm02 and θm) time series in all plots identify 126 (from a total of 7,615) data points that were flagged as suspect (+) and 25 data points that failed (o) quality control tests (Table 5).
A wave power time-series plot [Power] showing the total incident and alongshore wave power over the duration of each deployment, where alongshore power is relative to the average orientation of the shoreline adjacent to the deployment, which is provided in each deployment readme file.
Two wave rose plots based on the mean (θm) [RoseDm] and peak (θp) [RoseDp] wave directions. Fail data points (Table 5) are omitted from the wave rose plots.
A sea surface temperature plot [SeaTemp] showing water temperature measured by the wave buoy (only included if the instruments deployed had temperature sensors).
A readme file [Readme] (TXT format) is also provided and contains additional details about individual buoy service deployments and the entire deployment set for that location. Buoy deployment and retrieval dates, first and last data (recorded) dates, and mooring centroids and water depths (from high-resolution seabed mapping, see Usage Notes) are provided for each service deployment and for the entire deployment set, along with the total number of Qflag/Qcode value occurrences in the entire deployment dataset (Table 5).
A shapefile of buoy deployment locations for use in Geographical Information Systems (GIS) is also provided on the SEED portal dataset page57 and will be updated as new deployment data packages are added.
Real-time data received via satellite transmission from active wave buoy deployments can be viewed and downloaded from the SEED portal dataset page58 (see instructions below).
A rolling 7-day window of key wave parameters42 (Hm0, Tm01, Tp, θm, θp; Table 4), inferred wind speed and direction estimated from the wave spectra43, and surface water temperature (Spotter-v2/v3 deployments only) are provided for each active deployment in charts and data tables at half-hour intervals. The data are computed onboard the buoys following the manufacturers methods and are transmitted via satellite or cellular telemetry. They are not quality assessed or controlled in any way. Various factors may cause erroneous data points and users are advised to exercise caution when using the data. Real-time data are provided for information purposes and should not be solely relied upon for coastal hazard advice or to guide operational activities.
Select Map Viewer from the SEED portal dataset58 to open a Geocortex SEED Map and browse active wave buoy deployment locations, view real-time wave parameter time series data plots and tables, and download tabulated time series data. Real-time chart and table data can be browsed by clicking on a buoy location marker, selecting ‘View additional details’ in the data summary pop-up window, and then selecting ‘Show Expanded View’ from the drop-down menu list to change the side bar to landscape view. Data plots can be browsed using the ‘Charts’ tab and tabulated data using the buoy data type tabs.
Advanced users interested in wave buoy displacement data, wave spectra data and parameter time series data from the NSW nearshore wave buoy program can access the data in netCDF format via the THREDDS catalog on the Australian National Wave Archive59. Open the ‘NetCDF files via THREDDS catalog (NSW-DPE)’ link on the National Wave Archive dataset page to browse netCDF data by type and deployment location. The netCDF data are in the same format as the static dataset55 but will be updated upon the completion of underway (Table 3) and future deployments.
The WAVE_RAW_DISPLACEMENTS (buoy displacement) netCDF data fields include: TIME (displacement measurement time), TIME_LOCATION (location measurement time), LATITUDE (WGS84), LONGITUDE (WGS84), XDIS (east displacement), YDIS (north displacement) and ZDIS (heave displacement).
The WAVE_SPECTRA (spectral data) netCDF data fields include: TIME (half-hourly measurement interval), FREQUENCY (measurement frequency), LATITUDE (WGS84), LONGITUDE (WGS84), A1 (first term of Fourier cosine series), A2 (second term of Fourier cosine series), B1 (first term of Fourier sine series), B2 (second term of Fourier sine series) and ENERGY (energy density).
The WAVE_PARAMETERS (parameter data) netCDF data fields are indicated in square brackets in Table 4. All time fields in the netCDF data are expressed in days since 1/1/1950 00:00:00 UTC.
The accuracy of in-situ wave measurements using moored buoys, including GNSS buoys, has been investigated in laboratory and field studies and reported elsewhere61,62,63,64,65. The wave buoys used in this program measure their displacement using satellite positioning receivers and do not require calibration following manufacture as they do not feature any mechanical sensors. While the influences of the mooring on buoy motion cannot be completely avoided in all conditions, our moorings are fit for purpose and follow the manufacturer’s recommendations for shallow deployments (p. 95)41. The nearshore locations of our deployments are not typically subject to strong and sustained currents that may influence mooring behaviour at offshore deployment sites. Our data processing methods for spectral and time-domain derivation of wave parameters from surface buoy displacement data follow well-established techniques45,46,47 that are used by the buoy manufacturers for onboard data processing41,42.
Biofouling is a pervasive factor that is known to influence mooring behaviour and buoy motion when the fouling compromises the buoyancy properties of the mooring, which may lead to damping of high frequencies and/or introduce artefacts at low frequencies due to increased drag66,67,68,69. We observe mooring behaviour and buoy motion upon arriving at each deployment during service visits and retrievals to assess if the mooring might be compromised and inspect fouling upon removing equipment from the water. On the few occasions when heavy fouling due to a delayed service visit has been assessed as potentially impairing buoy motion (e.g., Fig. 2c,d), the processed data has been compared with concurrent data from the nearest offshore wave buoy (Fig. 1) and scrutinised for any changes in parameter behaviour (e.g., damping) through the deployment period. To date, this has resulted in truncation of affected datasets from the Collaroy 3 and Merimbula deployments (see Data loss and omission). Data from a heavily fouled deployment at Boomerang B shown in Fig. 2c was not affected as the batteries in the Datawell buoy had run flat prior to heavy fouling.
We compared wave data measured by nearshore wave buoys at Stockton, Figure Eight Pools and Broulee with concurrent deep-water wave data from the nearest offshore wave buoys at Sydney, Wollongong and Batemans Bay respectively (Fig. 1). Those nearshore deployment locations each had more than one year of observations and cover a range of settings, including: an embayed southern corner in 13 m water depth (Stockton), an open coast in 30 m water depth (Figure Eight Pools), and an embayed northern corner in 13 m water depth (Broulee).
Scatter and quantile-quantile plot comparisons of Hm0 between the nearshore-offshore wave buoy pairs capture the varying influence of wave transformation processes on wave height attenuation from deep to shallow water between sites (Fig. 4). In all cases, wave heights are reduced from deep water (offshore buoy) to shallow water (nearshore buoy), consistent with expected wave attenuation. The greater scatter for the embayed Stockton and Broulee sites relative to Figure Eight Pools suggests higher sensitivity of wave height attenuation to other characteristics of the wave field (e.g., wave period and direction), in embayed settings. The lower wave height attenuation at Figure Eight Pools is consistent with the steep bathymetry and open coastline there, and the 30 m deployment water depth at that site (Fig. 1).
Scatter plots (left) and quantile-quantile plots (right) comparing significant wave height (Hm0) measured concurrently by nearshore wave buoys (NSB) and offshore wave buoys (OSB) at: (a,b) Stockton (13 m depth) and Sydney (90 m depth); (c,d) Figure Eight Pools (30 m depth) and Wollongong (80 m depth); and, (e,f) Broulee (13 m depth) and Batemans Bay (65 m depth). See Fig. 1 for NSB and OSB locations and Tables 1,2 for NSB deployment details.
The offshore-nearshore wave height comparisons demonstrate that the nearshore wave buoy deployments are capturing patterns of deep- to shallow-water wave transformation as would be expected from consideration of the deployment settings and emphasise the importance of the nearshore wave buoy data for understanding local wave climates for coastal research and management applications.
Deployments at our first eight locations were commenced and completed using Datawell buoys (Table 1). During the concurrent deployments at Farquhar and Old Bar in 2018 (Fig. 1), we carried out a direct comparison between the Datawell DWR-G4 buoy at Old Bar and two new Spotter-v1 buoys, to assess whether the wave data measured by the two instruments could be considered equivalent for our purposes. A Spotter buoy was deployed at Old Bar B, 100 m to the south of the Datawell buoy at Old Bar A in similar water depth (Table 2). The paired Old Bar deployments were maintained for two months (3 October-4 December 2018), with a service visit on 23 October to change the Datawell buoy batteries and swap the Spotter buoy (to test both new Spotters). At the end of the comparison, the Datawell buoy at Old Bar A was removed (Table 1) and the Spotter deployment was maintained at Old Bar B until 11 March 2019 (Table 2). We processed the displacement data collected by the adjacent Datawell and Spotter buoys using uniform methods as previously described, see Methods, (Data processing).
The time series of concurrent wave data over the 2-month comparison period indicates close agreement between key wave height, period and direction parameters measured by the two instruments, showing consistency for most conditions and some notable deviations (Fig. 5). The Datawell buoy tended to predict higher significant wave height (Hm0) during energetic conditions, which were often accompanied by spikes in peak period (Tp). Such data points (red circles in Fig. 5) typically failed diagnostic tests (Qflag 4) and emerge from artefacts in the displacement data that are further discussed below (see Erroneous data). Mean wave period (Tm02) and mean wave direction (θm) compared well with minor deviation on some occasions when Hm0 measured by the Datawell buoy crept above that measured by the Spotter buoy. Wave roses generated from the Datawell and Spotter data (not shown here) were very consistent.
Measured wave height (a), period (b) and direction (c) data from the 2-month Datawell-Spotter buoy comparison at Old Bar (Fig. 1c), where Spotter buoys were deployed at Old Bar B (Table 2), 100 m south of the concurrent Datawell deployment at Old Bar A (Table 1). Circle markers on the Hm0 time series identify Datawell (red) and Spotter (blue) data points that failed quality control tests (Qflag 4, Table 5). Also shown are (d) wind speeds (average and gust) measured by anemometer at Port Macquarie airport (66 km north of Old Bar) during the deployments.
Scatter and quantile-quantile plots provide a more informative comparison of wave data recorded by the Datawell and Spotter buoys (Fig. 6). Buoy data pairs for timestamps where either Datawell or Spotter data failed quality control tests (marked by circles in Fig. 5) were omitted from the statistical comparisons. The positive bias in Hm0 for the Datawell buoy is evident for larger wave heights, however, inspection of Fig. 5 shows that for instances of Hm0 > 2 m when data passed quality control tests, measured wave heights were similar. In comparison, Tm02 and θm compare very well across the observed ranges. The positive bias in Datawell peak periods for Tp > 14 s derives from the varying frequency resolution between the two instruments, which is evident in the Tp scatter plot. Oscillation in spectral peak parameters for conditions with multiple energy spectrum peaks makes comparisons of Tp only generally informative. Table 6 provides standard comparison statistics describing the closeness of fit between wave parameters measured by the Datawell and Spotter buoys at Old Bar and shows similar consistency for spectral and time-domain parameters.
Scatter plots (left) and quantile-quantile plots (right) comparing significant wave height (Hm0; a,b), mean wave period (Tm02; c,d), peak wave period (Tp; e,f) and mean wave direction (θm; g,h), measured by Datawell (DW) and Spotter (SPOT) wave buoys located 100 m apart at Old Bar A and Old Bar B respectively (Fig. 1c), during the 2-month comparison deployments (Fig. 5). Data pairs for timestamps at which Datawell or Spotter data failed quality control tests (marked by red and blue circles respectively in Fig. 5) were omitted from the data comparison plots. Data comparison statistics are provided in Table 6.
Considering the complex bathymetry at Old Bar (Fig. 1), minor variations in wave parameters measured by the Datawell and Spotter buoys might be expected due to random wave fields and fine-scale variability in wave transformation in that setting. The closeness of fit between the two datasets thus demonstrates that the Spotter buoy provides comparable in-situ wave observations to the Datawell buoy, and the two measurement platforms can be considered equivalent for the purposes of our program. The results of our buoy comparison experiment are consistent with those previously reported that document strong agreement between parametric wave data from measurements made by Datawell DWR-G and Spotter GNSS buoys, and also motion sensor buoys (e.g., Datawell DWR-MkIII and DWR-4, TriAXYS)51,70,71,72,73. The key difference between data collected using Datawell DWR-G4 and Spotter buoys in our program appears to be in the occurrence and nature of artefacts in the recorded displacement data that lead to erroneous wave spectra and derived wave parameters.
A weakness of GNSS wave buoys that use a satellite receiver to measure their displacement, rather than internal motion sensors, is that measurements can be affected when the satellite receiver is obstructed, for example by saltwater overtopping during collision of unbroken or breaking sea waves, or simply if insufficient satellites are in range to accurately measure buoy displacement using Doppler shift or position data48,51,61,63. For this reason, the Datawell DWR-G4 buoy is only rated for moored deployments in currents less than 1 m/s, as the “bow wave” of the buoy under drag could overtop the hull41. In Datawell GPS buoy displacement data, an artefact caused by satellite data interruption manifests as a sawtooth pattern and introduces erroneous low-frequency energy into derived wave spectra48,49,50,51. The artefact is not attributed to the mooring influence (although that might exacerbate it) but derives from temporary disruption to the satellite data stream. The Datawell satellite receiver accesses a smaller pool of GPS satellites than Spotter GNSS buoys, which operate on the Iridium satellite network.
We compared wind data from an anemometer at Port Macquarie Airport (66 km north from Old Bar) with measured wave data during the Old Bar buoy comparison experiment (Fig. 5) to investigate if fail data points (Qflag 4) deriving from Datawell and Spotter displacement data artefacts correlated with above average winds. Above-average wind speeds were experienced prior to and/or during most of the 24 and 7 instances of fail data (Qcode 5) in the Datawell and Spotter records respectively. A complete displacement record (Percent = 100) was stored on the data cards for all instances of fail data, and thus the erroneous data points were caused by artefacts in the displacement data rather than insufficient displacement data. For notable occurrences of high wind speeds but no fail wave data points, wind directions were found to play a role. For example, wind speeds exceeded 18 knots with gusts to 30 knots on 8/11/2018, however, wind directions were from the south-west (230–250° TN), meaning there was minimal fetch at Old Bar between the shore and the wave buoys (Fig. 1). While only a qualitative analysis, the comparison between wave buoy data and coastal wind observations suggests that the displacement artefacts experienced by both buoys may occur during above-average winds, and thus are likely caused by saltwater overtopping of buoys during short-sea conditions.
Strong winds and choppy seas do not always compromise the performance of GNSS buoys, however. For example, during 16–17 July 2021, strong WNW winds generated choppy sea at Deeban over a 2 km fetch (Fig. 1h), with real-time data from the buoy showing Hm0 > 0.5 m, Tm01 of 2-4 s and WNW wave directions (i.e., waves approaching from within the estuary rather than from offshore). Concurrent swell conditions measured at the Sydney offshore wave buoy (Fig. 1) were benign (<1 m with low-moderate period) and a nearby anemometer at Kurnell (10 km north-east of Deeban) recorded average wind speeds exceeding 30 knots with gusts over 40 knots. Surface wind speeds at the Deeban buoy (inferred by Sofar Ocean from the calculated wave spectra) were 15–20 knots. Throughout that period, wave data recorded by the Deeban Spotter buoy was unaffected, with no spikes in parameter values associated with erroneous displacement. Our observations follow others48,49,50,51,61,63 in suggesting that Datawell and Spotter GNSS wave buoys can suffer displacement measurement errors due to saltwater obstruction of satellite receivers, which may compromise derived spectra and parameters. Our analysis suggests that conditions with short-sea waves over an underlying swell may increase the potential for wave overtopping of buoys due to irregular buoy motion, short-wave collision and increased wave breaking.
The Spotter buoy appears to be more robust to displacement artefacts caused by satellite data disruption than the Datawell buoy. This is evident in only 7 fail Spotter data points during the buoy comparison at Old Bar compared to 24 fail Datawell data points (Fig. 5). Spotter buoys are not immune from displacement artefacts, however. In the full 5 months of Spotter data collected at Old Bar B there were 25 fail data points, with most causing spikes in peak parameters and some also causing spikes in mean parameters (Fig. 3). Whereas artefacts in Datawell displacement data typically cause unrealistic spikes in peak parameters (Tp, θp), mean parameters (Hm0, Tm02, θm) are less conspicuously affected, but evidently deviate from the unaffected Spotter data. This is most evident during the storm wave conditions measured during 5–16 October in the buoy comparison deployments (Fig. 5). While artefacts in Spotter data are less common, they may cause prominent spikes in both peak and mean parameters, as occurred at Old Bar B on 4 January and 4 February 2019 (Fig. 3). Inspection of displacement data from the affected timestamps indicates that large spikes in mean parameters occurred when the amplitudes of artefacts were much higher (e.g., 20 times) than the average heave displacements recorded during the affected half hour. In comparison, artefacts in Datawell displacement data were usually only 2–5 times higher than average heave displacements.
We filter the displacement data recorded by both buoys using both a coarse spike filter and Butterworth filter to remove or minimise the influence of artefacts on derived wave spectra and parameters (see Methods, Data processing). Datawell also provides a manually operated Gap Repair tool to manage the issue, which uses an alternative digital filtering method48. We compared filtered displacement data and derived wave spectra following our methods with the same following application of the Datawell Gap Repair tool, comparing with concurrent Spotter data without artefacts as a benchmark. Our automated filtering methods provided equivalent or better results in removing or minimising displacement artefacts compared to application of the manually operated Gap Repair tool. The remnant influence of displacement artefacts are most evident in our processed parameter time series data as prominent spikes in peak parameters that occur more frequently in Datawell buoy data, although mean parameter values may also be affected in such instances. Our diagnostic tests described in the Methods (Quality control) identify all data points that may remain affected by displacement artefacts after data processing. Data users are advised to review suspect and fail data (using Qflag and Qcode values described in Table 5) and use such data with caution.
Minor data gaps in the parameter time series data tables (Qflag/Qcode 9) occur during deployment service visits when the wave buoy and mooring are removed from the water for a short period of time (1–2 hours) to retrieve onboard data and either clean or swap the buoy and mooring. Such occasions are documented in the deployment readme files and are evident in the data tables as a change in buoy serial number if the instrument was replaced. Minor data loss may also occur during periods of limited satellite availability or if the satellite receiver experiences issues. The Maroubra deployment, for example, includes a 46-hour period during which the buoy did not record any data at all.
More significant data loss has occurred in some instances due to buoy or mooring failure, or when service visits were delayed for operational reasons. For Datawell deployments, the battery life limitation (c. 30 days) resulted in some moderate data gaps of several days due to delayed service visits. A data gap of 15 days occurred in the Bronte deployment when a Datawell buoy malfunctioned mid-deployment and failed to record any more data. The same instrument ceased to function during the Fairy Meadow deployment resulting in 33 days of data loss there relative to the concurrent Woonona deployment (Table 1).
If the deployment position changed significantly between service deployments (particularly water depth), or if there was an extended break (months) during the deployment, a location may have multiple data packages corresponding to successive interrupted deployments at the same location. Some notable examples are described below. A Spotter buoy at Boomerang A (Fig. 1d) was initially deployed in 11.4 m water depth and the buoy broke free from its mooring during a storm on 4/6/2019 (DD/MM/YYYY) and washed up on the beach. A replacement buoy was deployed 50 days later in deeper water (13.3 m) and that deployment was named Boomerang A2 (Table 2). Similarly, the service visit of the concurrent Datawell buoy deployment (Table 1) at Boomerang B (Fig. 1d) was delayed after the loss of the Spotter at Boomerang A, resulting in a data gap of 36 days after the Datawell batteries ran flat during which the mooring fouled (Fig. 2c). A Spotter buoy was redeployed on 24/7/2019 and that deployment was named Boomerang B2 (Table 2). That mooring eventually dragged its anchor in strong currents on 13/1/2020 and travelled 2 km south, and the four days of compromised data have been omitted from that data record.
The Collaroy location also consists of multiple data packages. The initial Collaroy deployment was from 2/3/2016 to 17/5/2016 (Collaroy 1), concurrent with the Narrabeen deployment. A Datawell buoy was redeployed at Collaroy on 3/6/2016 in a similar location (Collaroy2), prior to a severe storm12, and was retrieved on 21/7/2016 (Table 1). A Spotter buoy was later deployed at a similar location on 21/10/2019, which was dragged out of position during a storm on 9/2/2020 and retrieved 7 weeks later on 31/3/2020 in a heavily fouled condition (Collaroy3). Seven weeks of data collected after the storm have been omitted due to significant change in the position (and water depth) of the mooring (Table 2), and heavy fouling that was deemed to influence buoy behaviour after the storm. A replacement Spotter buoy was deployed on 31/3/2020 (Collaroy 4) and was maintained for more than 1.5 years. Additional deployments (Collaroy 5 and Collaroy 6) have been made since with brief gaps between (Tables 2, 3).
For Spotter deployments, a delayed service visit is only problematic if the motion of the buoy becomes compromised due to biofouling, or the solar panels are compromised by growth and the battery runs flat. Both impairments were the case during the first service deployment at Merimbula (Table 2), where the last month of data (3/4/2021 to 10/5/2021) has been omitted from the dataset following our assessment that buoy motion was compromised during that period by heavy fouling from blue mussels (Fig. 2d). In that case, the service visit had been delayed (>6 months from initial deployment) and biofouling was unprecedented in our experiences due to a local abundance of blue mussels in the region. Indeed, the Broulee and Bengello buoys (Fig. 1i) had been deployed a week prior to the Merimbula buoy, and when visited a week after retrieval of the Merimbula buoy, both moorings and buoys at those sites were functioning normally with considerably less biofouling. Finally, a location may have multiple numbered data packages to enable the delivery of retrieved data from an ongoing deployment (e.g., Bengello, Collaroy).
Many applications of the wave buoy data may benefit from complementary datasets including high-resolution seabed bathymetry and landform (geomorphology) mapping, and deep-water wave data from the offshore wave buoy network (Fig. 1). Seabed bathymetry and landform mapping data cover the locations of all nearshore wave buoy deployments and will be useful for interpreting the nearshore wave data and for application in shallow-water wave models (e.g., bathymetry and bottom friction). These data are described below.
The Australian Wave Buoy Operations and Data Management Guidelines44 are available from Ocean Best Practices and provide further details on buoy deployment and wave data quality control methods used in our program. Additional information on wave buoy deployments and wave modelling tools from the NSW Nearshore Wave Data Program are available online24.
A seamless topography-bathymetry model covering the entire NSW coastline may also be of interest to users of the wave buoy data. The model provides 5-m spatial resolution elevation data extending from >200 m distance inland out to 30 m water depth on average (49 m maximum). The dataset derives from seamless airborne lidar topography-bathymetry surveys carried out during July-December 201817. Data covering the entire NSW coast can be browsed in an online Geocortex viewer and downloaded through the NSW SEED portal14.
The lidar bathymetry data have also been classified using spatial analysis techniques to derive detailed mapping of seabed landform features, including rocky reefs and sediment plains16,74. The seabed landforms mapping data can be browsed in an online Geocortex viewer and downloaded from the NSW SEED portal15.
Additional to lidar-derived seabed mapping covering the whole NSW coast, vessel-based multibeam echosounder surveys are being carried out to capture high-resolution coastal bathymetry data to 50–60 m water depths in selected sediment compartments17. Bathymetry and seabed landforms mapping data from those surveys is also available from the NSW SEED portal75.
Long-term offshore wave buoy deployments have been maintained at seven locations along the NSW coast for multiple decades13 (Fig. 1). Deep-water wave data from the offshore wave buoy network are available from the Australian National Wave Archive59 or by contacting Manly Hydraulics Laboratory.
All data preparation, processing and analysis, and the generation of plots and data tables were carried out using MATLAB. The code used to process buoy data from displacements to the wave parameter time series data presented here is provided with the data packages on the SEED portal in html format and can be viewed as they appear in MATLAB by anyone without access to that proprietary software.
O’Reilly, W. C., Olfe, C. B., Thomas, J., Seymour, R. J. & Guza, R. T. The California coastal wave monitoring and prediction system. Coast. Eng. 116, 118–132 (2016).
Ludka, B. C. et al. Sixteen years of bathymetry and waves at San Diego beaches. Sci. Data 6, 161 (2019).
Article CAS PubMed PubMed Central Google Scholar
Dean, R. G. & Dalrymple, R. A. Coastal Processes with Engineering Applications (Cambridge University Press, 2002).
Roelvink, D. & Reniers, A. A Guide to Modeling Coastal Morphology (World Scientific, 2009).
Jackson, D. W. & Short, A. D. Sandy Beach Morphodynamics (Elsevier Ltd., 2020).
Integrated Ocean Observing System (IOOS). A National Operational Wave Observation Platform. (NOAA and USACE, 2009).
Ribal, A. & Young, I. R. 33 years of globally calibrated wave height and wind speed data based on altimeter observations. Sci. Data 6, 1–16 (2019).
Durrant, T., Greenslade, D., Hemer, M. & Trenham, C. A Global Wave Hindcast Focussed on the Central and South Pacific. CAWCR Tech. Rep. 070 (Bureau of Meteorology, 2014).
Greenslade, D. et al. 15 priorities for wind-waves research: an Australian perspective. Bull. Am. Meteorol. Soc. 101, 446–461 (2020).
Power, H. E., Pomeroy, A. W. M., Kinsela, M. A. & Murray, T. P. Research priorities for coastal geoscience and engineering: a collaborative exercise in priority setting From Australia. Front. Mar. Sci. 8, 645797 (2021).
Kinsela, M. A., Morris, B. D., Linklater, M. & Hanslow, D. J. Second-pass assessment of potential exposure to shoreline change in New South Wales, Australia, using a sediment compartments framework. J. Mar. Sci. Eng. 5, 61 (2017).
Harley, M. D. et al. Extreme coastal erosion enhanced by anomalous extratropical storm wave direction. Sci. Rep. 7, 6033 (2017).
Article PubMed PubMed Central ADS Google Scholar
Manly Hydraulics Laboratory, NSW Government. NSW Ocean Wave Data Collection Program https://mhl.nsw.gov.au/Data-Wave (2023).
Department of Climate Change, Energy, the Environment and Water, NSW Government. NSW Marine LiDAR Topo-Bathy 2018 Geotif. SEED Portal https://datasets.seed.nsw.gov.au/dataset/marine-lidar-topo-bathy-2018 (2023).
Department of Climate Change, Energy, the Environment and Water, NSW Government. NSW seabed landforms derived from marine lidar data 2022. SEED Portal https://datasets.seed.nsw.gov.au/dataset/nsw-seabed-landforms-derived-from-marine-lidar-data-2022 (2023).
Linklater, M. et al. Techniques for classifying seabed morphology and composition on a subtropical-temperate continental shelf. Geosciences 9, 141 (2019).
Kinsela, M. A. et al. Mapping the shoreface of coastal sediment compartments to improve shoreline change forecasts in New South Wales, Australia. Estuaries Coast. 45, 1143–1169 (2022).
McLean, R., Thom, B., Shen, J. & Oliver, T. 50 years of beach–foredune change on the southeastern coast of Australia: Bengello Beach, Moruya, NSW, 1972–2022. Geomorphology 439, 108850 (2023).
Turner, I. L. et al. A multi-decade dataset of monthly beach profile surveys and inshore wave forcing at Narrabeen, Australia. Sci. Data 3, (2016).
Luijendijk, A. et al. The state of the world’s beaches. Sci. Rep. 8, 6641 (2018).
Article PubMed PubMed Central ADS Google Scholar
Vos, K., Splinter, K. D., Harley, M. D., Simmons, J. A. & Turner, I. L. CoastSat: a Google Earth Engine-enabled Python toolkit to extract shorelines from publicly available satellite imagery. Environ. Model. Softw. 122, 104528 (2019).
Robinet, A., Idier, D., Castelle, B. & Marieu, V. A reduced-complexity shoreline change model combining longshore and cross-shore processes: the LX-Shore model. Environ. Model. Softw. 109, 1–16 (2018).
Kinsela, M., Morris, B., Ingleton, T., Sutherland, M. & Hanslow, D. Nearshore wave observation and modelling to understand and manage coastal hazard risks. 2nd International Workshop on Waves, Storm Surges and Coastal Hazards (Melbourne, 2019).
Department of Climate Change, Energy, the Environment and Water, NSW Government. Ocean and Coastal Waves https://www.environment.nsw.gov.au/research-and-publications/our-science-and-research/our-research/water/ocean-and-coastal-waves (2023).
Taylor, D. et al. Verification of a coastal Wave Transfer Function for the New South Wales coastline. Australasian Coasts and Ports Conference (Auckland, 2015).
Garber, S., Taylor, D., Burston, J. & Dent. J. NSW Coastal Wave Model: State-Wide Nearshore Wave Transformation Tool. Report 12359.101.R2 (Baird Australia, 2017).
Stringari, C. E. & Power, H. E. Lidar observations of multi-modal swash probability distributions on a dissipative beach. Remote Sens. 13, 1–16 (2021).
Stockton Bight Sand Movement Study. Report No. P19028 (Bluecoast Consulting Engineers & City of Newcastle, 2020).
Power, H. E. et al. Automated sensing of wave inundation across a rocky shore platform using a low-cost camera system. Remote Sens. 10, 11 (2018).
Leaman, C. K. et al. A Storm Hazard Matrix combining coastal flooding and beach erosion. Coast. Eng. 170, 104001 (2021).
Short, A. D. Australian Coastal Systems: Beaches, Barriers and Sediment Compartments Coastal Research Library 32 (Springer, 2020).
Wright, L. D. Nearshore wave-power dissipation and the coastal energy regime of the Sydney-Jervis Bay region, New South Wales: a comparison. Aust. J. Mar. Freshwater Res. 27, 633–640 (1976).
Roy, P. S. & Thom, B. G. Late Quaternary marine deposition in New South Wales and southern Queensland - an evolutionary model. J. Geol. Soc. Aust. 28, 471–489 (1981).
Short, A. D. & Trenaman, N. L. Wave climate of the Sydney region, an energetic and highly variable ocean wave regime. Aust. J. Mar. Freshwater Res. 43, 765–791 (1992).
Harley, M. D., Turner, I. L., Short, A. D. & Ranasinghe, R. Interannual variability and controls of the Sydney wave climate. Int. J. Climatol. 30, 1322–1335 (2010).
Mortlock, T. R. & Goodwin, I. D. Directional wave climate and power variability along the Southeast Australian shelf. Cont. Shelf Res. 98, 36–53 (2015).
Shand, T. D. et al. NSW Coastal Inundation Hazard Study: Coastal Storms and Extreme Waves. Tech. Rep. 2010/16 (UNSW Water Research Laboratory, 2011).
Goodwin, I. D., Mortlock, T. R. & Browning, S. Tropical and extratropical-origin storm wave types and their influence on the East Australian longshore sand transport system under a changing climate. J. Geophys. Res. Ocean. 121, 4833–4853 (2016).
Datawell B.V. Directional Waverider DWR-G4 https://datawell.nl/products/directional-waverider-dwr-g4 (2023).
Sofar Ocean. Spotter: the agile metocean buoy https://www.sofarocean.com/products/spotter (2023).
Datawell Waverider Reference Manual: DWR-MkIII, DWR-G, WR-SG. (Datawell B.V., 2020).
Smit, P. B. Wave Parameter Definitions: Spoondrift (Sofar Ocean, 2018).
Voermans, J. J., Smit, P. B., Janssen, T. T. & Babanin, A. V. Estimating wind speed and direction using wave spectra. J. Geophys. Res. Ocean. 125, e2019JC015717 (2020).
Hansen, J. & Kinsela, M. Australian Wave Buoy Operations and Data Management Guidelines Manual. Version 1.0. Integrated Marine Observing System (IMOS), Ocean Best Practices https://repository.oceanbestpractices.org/handle/11329/2306, https://doi.org/10.26198/v2cn-f492 (2023).
Longuet-Higgins, M. S., Cartwright, D. E. & Smith, N. D. Observations of the directional spectrum of sea waves using the motions of a floating buoy. Ocean Wave Spectra: Proceedings of a Conference (Prentice-Hall, 1963).
Kuik, A. J., van Vledder, G. P. H. & Holthuijsen, L. H. A method for the routine analysis of pitch-and-roll buoy wave data. J. Phys. Oceanogr. 18, 1020–1034 (1988).
Holthuijsen, L. H. Waves in Oceanic and Coastal Waters (Cambridge University Press, 2007).
de Vries, J. J. Method and program to repair GPS gaps in DWR-G wave buoy data (extended version). Technical Note T23.01 (Datawell B.V., 2009).
Bjӧrkqvist, J. V. et al. Removing low-frequency artefacts from Datawell DWR-G4 wave buoy measurements. Geosci. Instrumentation, Methods Data Syst. 5, 17–25 (2016).
Boswood, P., Wall, R. & Rijkenberg, L. Monitoring and modelling extreme wave conditions during Tropical Cyclone Nathan. Australasian Coasts and Ports Conference (Cairns, 2017).
Andrews, E. & Peach L. Wave monitoring equipment comparison: An evaluation of current and emerging in-situ ocean wave monitoring technology. (Queensland Department of Environment and Science, 2019).
Ocean Data Standards - Volume 3: Recommendation for a Quality Flag Scheme for the Exchange of Oceanographic and Marine Meteorological Data. Report IOC/2013/MG/54-3 (Intergovernmental Oceanographic Commission, 2013).
Manual for Real-Time Quality Control of In-Situ Surface Wave Data – A Guide to Quality Control and Quality Assurance of In-Situ Surface Wave Observations. Version 2.1 (U.S. Integrated Ocean Observing System, 2019).
Manual for Real-Time Oceanographic Data Quality Control Flags. Version 1.2 (U.S. Integrated Ocean Observing System, 2020).
Kinsela, M. A. et al. NSW nearshore wave buoy data (2016-2023), Australian Ocean Data Network, https://doi.org/10.26198/jtsf-sh87 (2024).
Department of Climate Change, Energy, the Environment and Water, NSW Government. SEED: The Central Resource for Sharing and Enabling Environmental Data in NSW. SEED Portal https://www.seed.nsw.gov.au (2023).
Department of Climate Change, Energy, the Environment and Water, NSW Government. Wave parameter time series data (completed deployments). SEED Portal https://datasets.seed.nsw.gov.au/dataset/nsw-nearshore-wave-buoy-parameter-time-series-data-completed-deployments (2023).
Department of Climate Change, Energy, the Environment and Water, NSW Government. Wave parameter time series data (active deployments). SEED Portal https://datasets.seed.nsw.gov.au/dataset/nsw-nearshore-wave-buoy-parameter-time-series-data-active-deployments (2023).
Integrated Marine Observing System (IMOS) et al. Wave buoys Observations - Australia - delayed (National Wave Archive). Australian Ocean Data Network https://catalogue-imos.aodn.org.au/geonetwork/srv/eng/catalog.search#/metadata/2807f3aa-4db0-4924-b64b-354ae8c10b58 (2023).
Catching Oz Waves. National infrastructure for in-situ wave observations. Australian Research Data Commons https://doi.org/10.47486/DP748 (2023).
de Vries, J. J., Waldron, J. & Cunningham, V. Field tests of the new Datawell DWR-G GPS wave buoy. Sea Technol. 44, 50–55 (2003).
Janssen, P. A. E. M., Abdalla, S., Hersbach, H. & Bidlot, J. R. Error estimation of buoy, satellite, and model wave height data. J. Atmos. Ocean. Technol. 24, 1665–1677 (2007).
Herbers, T. H. C., Jessen, P. F., Janssen, T. T., Colbert, D. B. & MacMahan, J. H. Observing ocean surface waves with GPS-tracked buoys. J. Atmos. Ocean. Technol. 29, 944–959 (2012).
Joodaki, G., Nahavandchi, H. & Cheng, K. Ocean Wave Measurement Using GPS Buoys. J. Geod. Sci. 3, 163–172 (2013).
Liu, Q., Lewis, T., Zhang, Y. & Sheng, W. Performance assessment of wave measurements of wave buoys. Int. J. Mar. Energy 12, 63–76 (2015).
Frequency range and marine fouling, Technical Note T.39.01. https://datawell.nl/wp-content/uploads/2022/08/datawell_technicalnote_dwr4_fouling_highfrequency_t-39-01.pdf (Datawell B.V., 2012).
Ashton, I. G. C. & Johanning, L. On errors in low frequency wave measurements from wave buoys. Ocean Eng. 95, 11–22 (2015).
Thomson, J. et al. Biofouling effects on the response of a wave measurement buoy in deep water. J. Atmos. Ocean. Technol. 32, 1281–1286 (2015).
Campos, R. M., Islam, H., Ferreira, T. R. S. & Guedes Soares, C. Impact of heavy biofouling on a nearshore heave-pitch-roll wave buoy performance. Appl. Ocean Res. 107, 102500 (2021).
Raghukumar, K. et al. Performance characteristics of ‘spotter,” a newly developed real-time wave measurement buoy. J. Atmos. Ocean. Technol. 36, 1127–1141 (2019).
Andrews, E. & Peach L. An evaluation of current and emerging in-situ ocean wave monitoring technology. Australasian Coasts and Ports Conference (Hobart, 2019).
Lancaster, O., Cossu, R., Boulay, S., Hunter, S. & Baldock, T. E. Comparative wave measurements at a wave energy site with a recently developed low-cost wave buoy (Spotter), ADCP and pressure loggers. J. Atmos. Ocean. Technol. 38, 1019–1033 (2021).
Hansen, J. Cuttler, M., Lowe, R., Young, I. & Ierodiaconou, D. Displacement based comparison of accelerometer and low-cost GNSS wave buoys. 37th International Conference on Coastal Engineering (Sydney, 2022).
Linklater, M., Morris, B. D. & Hanslow, D. J. Classification of seabed landforms on continental and island shelves. Front. Mar. Sci. 10, 1258556 (2023).
Department of Climate Change, Energy, the Environment and Water, NSW Government. Seabed NSW. SEED Portal https://datasets.seed.nsw.gov.au/dataset/seabed-nsw (2023).
The NSW Nearshore Wave Buoy Program is operated by the Coastal and Marine Science team, Environment and Heritage, Department of Climate Change, Energy, the Environment and Water, NSW Government, with funding from the NSW Coastal Reforms and Climate Change Fund. The Integrated Marine Observing System (IMOS) NSW Node supported the purchase of some wave buoys and mooring equipment with funding from the Research Attraction and Acceleration Program (RAAP), Office of the NSW Chief Scientist and Engineer. The Sydney Institute of Marine Science (SIMS) managed IMOS-RAAP purchases and provided meeting spaces, a vessel and science communication services. Water Research Laboratory (UNSW) wave buoys deployed at Collaroy were funded by an Australian Research Council grant (ARCLP170100161) and internal funding led by Kristen Splinter. Manly Hydraulics Laboratory provided offshore wave buoy data and Datawell buoy painting. Coastal wind data were collected by the Bureau of Meteorology (BOM). NSW Roads and Maritime Services and Police Marine Area Command assisted with safety of navigation. NSW Department of Primary Industries (DPI) assisted with Marine Parks permits. We thank all who have assisted with wave buoy operations, including Tim Austin, Chris Drummond and Chris Leaman (UNSW), Gwenael Cadiou (DPI), and Hannah Power (UON). We thank Jeff Hansen (UWA) and Leo Peach (BOM) for useful discussions on methods, and Stuart Young (NSWENV) for reviewing the original manuscript.
Water, Wetlands and Coasts Science, Environment and Heritage, Department of Climate Change, Energy, the Environment and Water, NSW Government, Lidcombe, Australia
Michael A. Kinsela, Bradley D. Morris, Timothy C. Ingleton, Thomas B. Doyle, Michael D. Sutherland, Neil E. Doszpot, Jeff J. Miller, Stephen F. Holtznagel & David J. Hanslow
School of Environmental and Life Sciences, University of Newcastle, Callaghan, Australia
Water Research Laboratory, School of Civil and Environmental Engineering, UNSW, Manly Vale, Australia
You can also search for this author in PubMed Google Scholar
You can also search for this author in PubMed Google Scholar
You can also search for this author in PubMed Google Scholar
You can also search for this author in PubMed Google Scholar
You can also search for this author in PubMed Google Scholar
You can also search for this author in PubMed Google Scholar
You can also search for this author in PubMed Google Scholar
You can also search for this author in PubMed Google Scholar
You can also search for this author in PubMed Google Scholar
You can also search for this author in PubMed Google Scholar
M.A.K. the primary author, coordinated the wave buoy program and lead the manuscript preparation with input from B.D.M. M.A.K. and B.D.M. lead the data collection, equipment management, data processing, quality control and data delivery. T.C.I. and T.B.D. contributed to data collection, equipment and program management, and reviewed the manuscript. M.D.S., N.E.D., J.J.M. and S.F.H. contributed to data collection and equipment management. M.D.H. coordinated data collection at Collaroy and reviewed the manuscript. D.J.H. contributed to program management and reviewed the manuscript.
Correspondence to Michael A. Kinsela.
M.A.K., B.D.M., T.C.I., T.B.D., M.D.S., N.E.D., J.J.M., S.F.H. and D.J.H. were employed in marine scientist and technician roles by the Government of New South Wales, Australia.
Publisher’s note Springer Nature remains neutral with regard to jurisdictional claims in published maps and institutional affiliations.
Open Access This article is licensed under a Creative Commons Attribution 4.0 International License, which permits use, sharing, adaptation, distribution and reproduction in any medium or format, as long as you give appropriate credit to the original author(s) and the source, provide a link to the Creative Commons licence, and indicate if changes were made. The images or other third party material in this article are included in the article’s Creative Commons licence, unless indicated otherwise in a credit line to the material. If material is not included in the article’s Creative Commons licence and your intended use is not permitted by statutory regulation or exceeds the permitted use, you will need to obtain permission directly from the copyright holder. To view a copy of this licence, visit http://creativecommons.org/licenses/by/4.0/.
Kinsela, M.A., Morris, B.D., Ingleton, T.C. et al. Nearshore wave buoy data from southeastern Australia for coastal research and management. Sci Data 11, 190 (2024). https://doi.org/10.1038/s41597-023-02865-x
DOI: https://doi.org/10.1038/s41597-023-02865-x
Anyone you share the following link with will be able to read this content:
Sorry, a shareable link is not currently available for this article.
Provided by the Springer Nature SharedIt content-sharing initiative
Scientific Data (Sci Data) ISSN 2052-4463 (online)

Lake Wind Buoy Sign up for the Nature Briefing newsletter — what matters in science, free to your inbox daily.